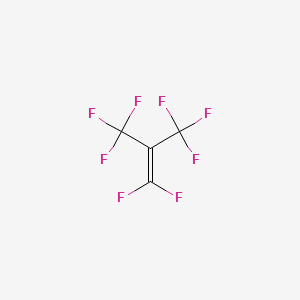
Perfluoroisobutylene
- Click on QUICK INQUIRY to receive a quote from our team of experts.
- With the quality product at a COMPETITIVE price, you can focus more on your research.
Overview
Description
Perfluoroisobutylene (PFIB, C₄F₈) is a highly toxic, colorless gas with a faint grassy odor. It is primarily generated as a decomposition product of polytetrafluoroethylene (PTFE or Teflon®) during pyrolysis at temperatures exceeding 300°C . PFIB is also a byproduct of industrial processes involving fluorinated fluids, such as vapor-phase soldering . Its extreme toxicity—10 times more lethal than phosgene—stems from its rapid hydrolysis in the lungs, producing reactive intermediates like fluorophosgene (COF₂) and hydrofluoric acid (HF), which cause acute pulmonary edema and oxidative stress .
Scientific Research Applications
Toxicological Research
Acute Lung Injury Studies
PFIB is notorious for causing acute lung injury (ALI) upon inhalation. Research has demonstrated that exposure to PFIB leads to significant pathological changes in lung tissues, including inflammation and apoptosis of lung cells. A study conducted on rats exposed to PFIB revealed that inflammatory cell infiltration and alveolar wall thickening occurred within hours of exposure, peaking at 24 hours before gradually recovering .
Key Findings:
- Mechanism of Injury : The injury mechanism involves oxidative stress and a cytokine storm, leading to increased levels of pro-inflammatory cytokines in lung tissues .
- Preventive Measures : Pretreatment with pyrrolidine dithiocarbamate has shown protective effects against PFIB-induced ALI by inhibiting NF-kappaB activation .
Analytical Chemistry
Detection and Analysis Using Metal-Organic Frameworks
PFIB's unique chemical properties have made it a subject of interest in analytical chemistry, particularly in the development of Metal-Organic Frameworks (MOFs). These frameworks are being researched for their potential to selectively detect PFIB and other harmful compounds due to their high porosity and tunable surface area .
Applications in Analytical Chemistry:
- Sample Preparation : MOFs can enhance the selectivity and sensitivity of detecting PFIB in various matrices.
- Environmental Monitoring : The ability to detect PFIB in environmental samples is crucial for assessing contamination levels from industrial processes.
Material Science
Development of Fluoropolymer Products
PFIB is also relevant in the production of fluoropolymers, which have applications across various industries due to their chemical resistance and thermal stability. Research into the synthesis of fluoropolymers from PFIB has potential implications for creating advanced materials used in medical devices, coatings, and seals.
Case Studies:
- Fluoropolymer Synthesis : Studies have explored the conversion of PFIB into useful fluoropolymer products through controlled polymerization processes .
- Medical Applications : The biocompatibility and non-reactive nature of fluoropolymers derived from PFIB make them suitable for use in medical implants and devices .
Summary Table of Applications
Q & A
Basic Research Questions
Q. What are the primary mechanisms of PFIB-induced pulmonary toxicity, and how are these pathways experimentally validated?
PFIB's acute toxicity arises from its reactivity with lung surfactants and alveolar epithelial cells, leading to pulmonary edema and inflammation. Experimental validation involves exposing rodent models to controlled PFIB concentrations (e.g., 0.5–5 ppm) and analyzing histopathological changes, bronchoalveolar lavage fluid (BALF) biomarkers (e.g., protein content, inflammatory cytokines), and oxidative stress markers. Key studies by Lehnert et al. (1993) demonstrated dose-dependent increases in lung permeability and neutrophil infiltration within 24 hours post-exposure . Smith et al. (1982) further correlated exposure duration with mortality rates in rats, establishing LC₅₀ values .
Q. How should researchers design inhalation toxicity studies for PFIB to ensure reproducibility?
Standardized protocols include:
- Exposure Systems : Dynamic airflow chambers with real-time gas concentration monitoring (e.g., infrared spectroscopy) .
- Control Groups : Sham-exposed animals and positive controls (e.g., phosgene) to benchmark toxicity .
- Endpoint Analysis : Histopathology (H&E staining), BALF analysis, and pulse oximetry for hypoxia assessment.
- Data Reporting : Adherence to the Beilstein Journal’s guidelines for experimental detail, including chamber specifications, exposure duration, and statistical methods .
Q. What analytical methods are recommended for detecting PFIB in environmental or laboratory air samples?
The U.S. EPA’s SAM Method 5.2.85 recommends gas chromatography (GC) coupled with flame photometric detection (FPD) or nitrogen-phosphorus detection (NPD). Thermal desorption is used for sample preparation, with a detection limit of 0.1 µg/m³. This method is validated for distinguishing PFIB from interferents like phosgene .
Advanced Research Questions
Q. How can conflicting data on PFIB’s concentration-response relationships be resolved in inhalation studies?
Discrepancies often stem from variability in exposure systems (e.g., static vs. dynamic chambers) or interspecies differences. A meta-analysis approach, as applied by Bide et al. (2000), can harmonize data by normalizing results to respiratory minute volumes and adjusting for chamber humidity/temperature . Computational toxicology models (e.g., physiologically based pharmacokinetic models) further reconcile differences by simulating species-specific alveolar deposition .
Q. What strategies optimize GC-MS protocols for PFIB quantification in complex matrices (e.g., combustion byproducts)?
Key optimizations include:
- Column Selection : Polar capillary columns (e.g., DB-624) to separate PFIB from co-eluting perfluorocarbons .
- Derivatization : Use of pentafluorobenzyl bromide to enhance ionization efficiency in electron-capture negative ion mode .
- Matrix Interference Mitigation : Solid-phase microextraction (SPME) for selective PFIB isolation from particulate matter .
Q. How do PFIB’s thermal decomposition pathways vary under different combustion conditions, and what are the implications for hazard assessment?
PFIB decomposes at >300°C into carbonyl fluoride (COF₂) and hydrogen fluoride (HF), but secondary reactions with metals (e.g., aluminum) produce trifluoroacetyl fluoride (CF₃COF). Warheit et al. (1990) showed that aerosol aging and combustion temperature (400–600°C) significantly alter byproduct profiles, necessitating thermogravimetric analysis (TGA) and FTIR spectroscopy for real-time monitoring .
Methodological and Data Analysis Questions
Q. What statistical frameworks are most robust for analyzing PFIB’s dose-dependent mortality data?
Probit analysis is widely used to calculate LC₅₀ values, but Bayesian hierarchical models improve accuracy in small-sample studies by incorporating prior toxicity data (e.g., from structurally similar perfluorocarbons) . For sublethal endpoints (e.g., inflammation), mixed-effects models account for intra-animal variability .
Q. How can computational toxicology predict PFIB’s toxicological endpoints when experimental data are limited?
Quantitative structure-activity relationship (QSAR) models, such as those in the EPA’s TEST suite, predict acute toxicity using PFIB’s electrophilicity index (ω = 10.2 eV) and octanol-water partition coefficient (log Kₒw = 3.1). Molecular dynamics simulations further elucidate its interactions with pulmonary surfactants .
Q. Ethical and Reproducibility Considerations
Q. What ethical safeguards are critical in PFIB in vivo studies given its extreme toxicity?
Protocols must include:
- Exposure Control : Closed-circuit systems with HEPA filtration to protect researchers .
- Humane Endpoints : Predefined criteria for euthanasia (e.g., >20% body weight loss, severe dyspnea) .
- Regulatory Compliance : Approval from institutional animal care committees (IACUC) and adherence to OECD Test Guidelines 403 .
Comparison with Similar Compounds
Comparison with Structurally Similar Fluorinated Compounds
PFIB’s toxicity and environmental behavior are best understood when compared to related fluorinated alkenes and gases. Below is a detailed analysis:
Toxicity Profile
Key Observations :
- PFIB is ~50,000x more toxic than TFE and ~2,000x more toxic than HFP in inhalation studies .
- Unlike phosgene, PFIB’s effects manifest rapidly (1–4 hours post-exposure), with mortality linked to Bcl-2/Bax-mediated apoptosis and oxidative stress .
Environmental Persistence and Sources
- PFIB : Generated during PTFE pyrolysis, industrial fluorocarbon processes, and combustion of fluoropolymers . Atmospheric half-life is short (~1 hour) due to reactivity with water vapor .
- HFP and TFE : Industrial feedstocks for fluoropolymer production; longer atmospheric persistence (weeks to months) due to resistance to hydrolysis .
- Phosgene : Primarily industrial (pesticides, plastics); persists longer in dry air but hydrolyzes rapidly in humid environments .
Mechanistic Divergences
While PFIB and phosgene both cause pulmonary edema, their mechanisms differ:
- PFIB hydrolyzes to COF₂ and HF, triggering cytokine cascades (e.g., TNF-α, IL-6) and apoptosis via Akt pathway suppression .
- Phosgene acylates alveolar proteins, impairing surfactant function and leading to delayed edema .
- HFP and TFE exhibit lower reactivity, with toxicity linked to metabolic byproducts (e.g., trifluoroacetic acid) rather than direct hydrolysis .
Preparation Methods
Pyrolysis of Octafluorocyclobutane
Reaction Mechanism and Conditions
Octafluorocyclobutane (c-C₄F₈), a cyclic dimer of tetrafluoroethylene (TFE), undergoes atmospheric-pressure pyrolysis to yield PFIB. The reaction proceeds via ring-opening and rearrangement mechanisms, producing PFIB as the primary product with a reported yield of 50% . The process typically operates at temperatures between 600–800°C , with residence times optimized to maximize PFIB formation while minimizing decomposition into smaller perfluorocarbons.
The reaction is summarized as:
c-C₄F₈Δ(CF₃)2C=CF₂+byproducts
Industrial and Experimental Data
Key parameters from large-scale experiments include:
Parameter | Value | Source |
---|---|---|
Temperature | 700–750°C | |
Pressure | Atmospheric | |
PFIB Yield | 50% | |
Major Byproducts | Hexafluoropropene |
This method is favored in industrial settings due to its scalability and predictable yields. However, the toxicity of PFIB necessitates stringent containment protocols during production .
Thermal Decomposition of Polytetrafluoroethylene (PTFE)
Mechanism and Byproduct Formation
PTFE, a common fluoropolymer, decomposes at temperatures exceeding 450°C , releasing PFIB as a minor product alongside other perfluoroalkenes and carbonyl fluorides . The degradation follows a radical chain mechanism, initiated by homolytic cleavage of C–C bonds in the polymer backbone:
(CF₂–CF₂)nΔ(CF₃)2C=CF₂+CF₂=CF₂+COF₂
Thermolysis of Chlorofluorocarbons (CFCs)
HCFC-124 and HCFC-22 as Feedstocks
Thermolysis of hydrochlorofluorocarbons (HCFCs), such as HCFC-124 (CF₃CF₂Cl) and HCFC-22 (CHClF₂), produces PFIB as a secondary product. For example, pyrolysis of HCFC-124 at 700–850°C yields PFIB at concentrations below 0.1% , with hexafluoropropene (HFP) as the major product .
Table 1: Thermolysis of HCFC-124 at Varying Conditions
Temperature (°C) | Contact Time (s) | PFIB Yield (%) | Major Product |
---|---|---|---|
700 | 2.5 | <0.01 | HFP (62%) |
815 | 1.8 | 0.05 | HFP (58%) |
850 | 1.5 | 0.08 | HFP (55%) |
Role of Catalysts and Reactor Design
The use of platinum-lined reactors and inert gas purging reduces PFIB formation by suppressing radical recombination . For instance, introducing perfluorocyclobutane (c-C₄F₈) as a co-feedstock in HCFC-124 thermolysis lowers PFIB yields to <0.01% .
Comparative Analysis of Preparation Methods
Yield and Scalability
Properties
CAS No. |
382-21-8 |
---|---|
Molecular Formula |
C4F8 |
Molecular Weight |
200.03 g/mol |
IUPAC Name |
1,1,3,3,3-pentafluoro-2-(trifluoromethyl)prop-1-ene |
InChI |
InChI=1S/C4F8/c5-2(6)1(3(7,8)9)4(10,11)12 |
InChI Key |
DAFIBNSJXIGBQB-UHFFFAOYSA-N |
SMILES |
C(=C(F)F)(C(F)(F)F)C(F)(F)F |
Canonical SMILES |
C(=C(F)F)(C(F)(F)F)C(F)(F)F |
boiling_point |
0.5 °C 7 °C 44.6°F |
Color/Form |
Colorless gas Gas at room temperature |
density |
1.592 g/cu cm at 0 °C 1.6 g/l 1.592 |
melting_point |
-130 °C -202°F |
Key on ui other cas no. |
382-21-8 |
physical_description |
COLOURLESS GAS. Colorless, odorless gas at room temp. When heated to decomposition it emits toxic fumes of hydrogen fluoride. |
Pictograms |
Acute Toxic; Health Hazard |
solubility |
Decomposes in wate |
Synonyms |
perfluoroisobutene perfluoroisobutylene |
vapor_density |
6.3 (Air = 1) 6.3 |
vapor_pressure |
1740 mm Hg at 25 °C 1740 mmHg |
Origin of Product |
United States |
Disclaimer and Information on In-Vitro Research Products
Please be aware that all articles and product information presented on BenchChem are intended solely for informational purposes. The products available for purchase on BenchChem are specifically designed for in-vitro studies, which are conducted outside of living organisms. In-vitro studies, derived from the Latin term "in glass," involve experiments performed in controlled laboratory settings using cells or tissues. It is important to note that these products are not categorized as medicines or drugs, and they have not received approval from the FDA for the prevention, treatment, or cure of any medical condition, ailment, or disease. We must emphasize that any form of bodily introduction of these products into humans or animals is strictly prohibited by law. It is essential to adhere to these guidelines to ensure compliance with legal and ethical standards in research and experimentation.