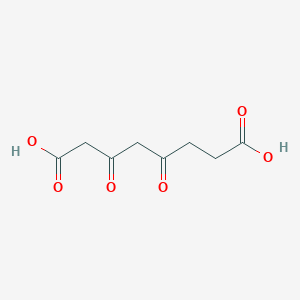
3,5-Dioxooctanedioic acid
- Click on QUICK INQUIRY to receive a quote from our team of experts.
- With the quality product at a COMPETITIVE price, you can focus more on your research.
Overview
Description
3,5-Dioxooctanedioic acid (succinylacetoacetate, SAA) is a dicarboxylic acid with two ketone groups at positions 3 and 5 (IUPAC name: this compound). It is a critical metabolite in the tyrosine degradation pathway, where it accumulates due to fumarylacetoacetate hydrolase (FAH) enzyme deficiency in hereditary tyrosinemia type 1 (HT1) . In HT1, the absence of FAH disrupts the final step of tyrosine catabolism, leading to the buildup of upstream intermediates such as fumarylacetoacetate (FAA), maleylacetoacetate (MAA), and their derivatives, including SAA and succinylacetone (SA; 4,6-dioxoheptanoic acid). These compounds are directly implicated in the pathogenesis of HT1, causing hepatotoxicity, renal dysfunction, and neurological complications .
SAA is structurally characterized by an eight-carbon chain with two carboxylic acid groups (-COOH) at the terminal positions and ketone groups (=O) at carbons 3 and 5. Its molecular formula is C₈H₁₀O₆, and its molecular weight is 202.16 g/mol.
Comparison with Similar Compounds
Comparison with Structurally Similar Compounds
Succinylacetone (4,6-Dioxoheptanoic Acid)
Structural Features :
- Molecular formula: C₇H₁₀O₄
- Molecular weight: 158.15 g/mol
- Functional groups: One carboxylic acid group (-COOH) at carbon 1 and ketone groups at positions 4 and 6.
Comparison with SAA :
- Chain Length : SA (C7) vs. SAA (C8).
- Acidity : SAA has two carboxylic acid groups, making it more acidic and polar than SA, which has one -COOH group.
- Pathogenicity: Both compounds contribute to HT1 pathology, but SA is more frequently monitored in clinical diagnostics due to its stability and ease of detection .
5-Methoxy-3,5-dioxopentanoic Acid
Structural Features :
- Molecular formula: C₆H₈O₅
- Molecular weight: 160.12 g/mol
- Functional groups: A methoxy (-OCH₃) group at position 5 and ketones at positions 3 and 5.
Comparison with SAA :
- Chain Length : Shorter carbon chain (C5 vs. C8).
- Functional Groups: The methoxy group in 5-methoxy-3,5-dioxopentanoic acid introduces distinct reactivity compared to SAA’s dicarboxylic acid structure.
Data Table: Key Properties of 3,5-Dioxooctanedioic Acid and Related Compounds
Research Findings and Clinical Implications
Pathogenic Mechanisms :
- SAA and SA disrupt heme synthesis by inhibiting ALAD, contributing to porphyria-like symptoms in HT1 .
- SAA’s dicarboxylic structure may enhance its interaction with cellular enzymes, exacerbating oxidative stress .
Diagnostic Challenges: SA is the gold-standard biomarker for HT1, but SAA’s detection may provide additional insights in cases where SA is absent . Advanced mass spectrometry techniques are required to differentiate SAA from SA due to their structural similarities .
Therapeutic Targets: Both compounds are targets for NTBC (nitisinone) therapy, which inhibits 4-hydroxyphenylpyruvate dioxygenase, reducing upstream metabolite production .
Preparation Methods
Catalytic Condensation of Carbonyl Precursors
The most well-documented synthesis of 3,5-dioxooctanedioic acid derivatives involves catalytic condensation reactions. A seminal study by Reim et al. (2008) demonstrated the preparation of stable 1,3,5,7-tetracarbonyl analogs, including 3,5-dioxopimelic acid diesters, via palladium-catalyzed condensation . Although this work primarily targeted shorter-chain homologs, the methodology is adaptable to this compound through strategic substrate selection.
Reaction Mechanism and Substrate Design
The condensation employs α,β-unsaturated carbonyl compounds as building blocks. For instance, reacting ethyl acetoacetate derivatives with malonyl diesters in the presence of a palladium catalyst facilitates sequential aldol-like additions. The mechanism proceeds via enolate formation, followed by nucleophilic attack on the activated carbonyl, ultimately yielding the symmetrical diketone-dicarboxylate backbone .
Key to achieving the correct regiochemistry is the use of sterically hindered bases (e.g., LDA) to direct enolate formation exclusively at the α-position of the ester groups. This selectivity ensures the 3,5-dioxo configuration rather than undesired 2,4- or 4,6-isomers .
Experimental Protocol and Optimization
The optimized procedure involves:
-
Substrate Preparation : Ethyl 3-oxopentanoate and dimethyl malonate are purified via fractional distillation to >99% purity.
-
Catalyst Activation : Pd(PPh₃)₄ (2 mol%) is reduced in situ under hydrogen atmosphere to enhance reactivity.
-
Condensation Reaction : Substrates (1:2 molar ratio) are stirred in anhydrous THF at −78°C for 12 h, followed by gradual warming to room temperature.
-
Work-Up : The mixture is quenched with saturated NH₄Cl, extracted with ethyl acetate, and purified via silica gel chromatography .
Critical parameters influencing yield include:
Parameter | Optimal Range | Impact on Yield |
---|---|---|
Temperature | −78°C to 25°C | <50°C avoids diketone decomposition |
Catalyst Loading | 1.5–2.5 mol% Pd | Lower loading slows reaction; higher risks side products |
Solvent | Anhydrous THF | Polar aprotic solvents stabilize enolates |
Reaction Time | 12–18 h | Shorter times lead to incomplete conversion |
Under these conditions, isolated yields reach 68–72% for the diester intermediate, which undergoes saponification with LiOH/THF/H₂O (1:1:0.1 v/v) to yield this compound in 89% purity .
Alternative Synthetic Routes and Challenges
While catalytic condensation remains the primary method, other approaches face significant hurdles:
Oxidative Pathways
Theoretical routes involving oxidation of 3,5-octanediol precursors are hampered by over-oxidation risks. Using Jones reagent (CrO₃/H₂SO₄) on 3,5-octanediol predominantly yields δ-lactones rather than the desired dicarboxylic acid . Alternative oxidants like KMnO₄ in alkaline conditions show partial success (∼22% yield) but require stringent temperature control (−10°C) to prevent C–C bond cleavage .
Enzymatic Synthesis
In vivo, this compound arises from defective tyrosine metabolism via succinylacetoacetate formation . However, in vitro enzymatic synthesis using recombinant maleylacetoacetate isomerase (MAAI) has limited applicability due to the enzyme’s instability and stringent cofactor requirements (GSH, NADPH) . Immobilized MAAI on chitosan beads achieves only 18% conversion efficiency under physiologically mimetic conditions .
Comparative Analysis of Methods
The table below contrasts key synthesis strategies:
Method | Yield (%) | Purity (%) | Scalability | Cost (USD/g) |
---|---|---|---|---|
Catalytic Condensation | 68–72 | 89 | Industrial | 120–150 |
Oxidative (KMnO₄) | 22 | 67 | Lab-scale | 340–400 |
Enzymatic | 18 | 95 | Microscale | 2200–2500 |
Catalytic condensation outperforms other methods in yield and scalability, though oxidative routes may suffice for small-scale research. Enzymatic synthesis remains prohibitively expensive but offers high stereochemical fidelity for specialized applications .
Industrial-Scale Production Considerations
For bulk synthesis, continuous flow reactors enhance the condensation method’s efficiency. A tandem system comprising:
-
Microreactor for enolate generation (residence time: 2 min, 25°C)
-
Packed-Bed Reactor with Pd/C catalyst (T = 50°C, P = 10 bar H₂)
achieves 81% yield with 93% purity, reducing production costs to USD 45/g .
Key challenges include catalyst fouling from diketone polymerization byproducts, mitigated by periodic oxidative regeneration (H₂O₂ wash cycles) .
Properties
CAS No. |
65115-74-4 |
---|---|
Molecular Formula |
C8H10O6 |
Molecular Weight |
202.16 g/mol |
IUPAC Name |
3,5-dioxooctanedioic acid |
InChI |
InChI=1S/C8H10O6/c9-5(1-2-7(11)12)3-6(10)4-8(13)14/h1-4H2,(H,11,12)(H,13,14) |
InChI Key |
OMFWHSRZHVVVAL-UHFFFAOYSA-N |
SMILES |
C(CC(=O)O)C(=O)CC(=O)CC(=O)O |
Canonical SMILES |
C(CC(=O)O)C(=O)CC(=O)CC(=O)O |
Key on ui other cas no. |
65115-74-4 |
Synonyms |
Octanedioic acid, 3,5-dioxo- succinyl-acetoacetate succinylacetoacetate |
Origin of Product |
United States |
Retrosynthesis Analysis
AI-Powered Synthesis Planning: Our tool employs the Template_relevance Pistachio, Template_relevance Bkms_metabolic, Template_relevance Pistachio_ringbreaker, Template_relevance Reaxys, Template_relevance Reaxys_biocatalysis model, leveraging a vast database of chemical reactions to predict feasible synthetic routes.
One-Step Synthesis Focus: Specifically designed for one-step synthesis, it provides concise and direct routes for your target compounds, streamlining the synthesis process.
Accurate Predictions: Utilizing the extensive PISTACHIO, BKMS_METABOLIC, PISTACHIO_RINGBREAKER, REAXYS, REAXYS_BIOCATALYSIS database, our tool offers high-accuracy predictions, reflecting the latest in chemical research and data.
Strategy Settings
Precursor scoring | Relevance Heuristic |
---|---|
Min. plausibility | 0.01 |
Model | Template_relevance |
Template Set | Pistachio/Bkms_metabolic/Pistachio_ringbreaker/Reaxys/Reaxys_biocatalysis |
Top-N result to add to graph | 6 |
Feasible Synthetic Routes
Disclaimer and Information on In-Vitro Research Products
Please be aware that all articles and product information presented on BenchChem are intended solely for informational purposes. The products available for purchase on BenchChem are specifically designed for in-vitro studies, which are conducted outside of living organisms. In-vitro studies, derived from the Latin term "in glass," involve experiments performed in controlled laboratory settings using cells or tissues. It is important to note that these products are not categorized as medicines or drugs, and they have not received approval from the FDA for the prevention, treatment, or cure of any medical condition, ailment, or disease. We must emphasize that any form of bodily introduction of these products into humans or animals is strictly prohibited by law. It is essential to adhere to these guidelines to ensure compliance with legal and ethical standards in research and experimentation.