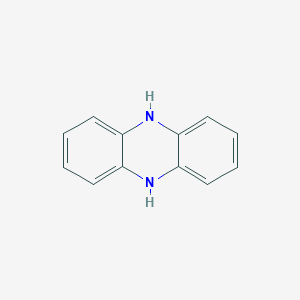
5,10-Dihydrophenazine
Overview
Description
1.1 Chemical Structure and Synthesis
5,10-Dihydrophenazine (C₁₂H₁₀N₂, molecular weight 182.226) is a partially hydrogenated derivative of phenazine, characterized by two hydrogen atoms at the 5- and 10-positions of the central heterocyclic ring . Early syntheses involved copper-catalyzed cross-coupling reactions, such as Gilman and Dietrich’s 1957 method using phenyllithium and phenazine . Modern approaches include palladium-catalyzed Buchwald–Hartwig double-arylation for symmetrical 5,10-diaryl derivatives and sequential N-arylation for unsymmetrical derivatives . Iron-catalyzed intramolecular reactions have also been employed to synthesize 2,7-disubstituted derivatives, though yields vary depending on substituents (e.g., 20–35% for halogenated substrates) .
1.2 Physicochemical Properties
The compound exhibits a density of 1.152 g/cm³, melting point of ~358.5°C, and redox-active behavior due to its conjugated π-system . Its reduced state (dihydrophenazine) and oxidized state (phenazine) enable reversible electron transfer, critical for applications in photoredox catalysis and energy storage .
Preparation Methods
Solvent-Mediated Condensation of Catechols and o-Phenylenediamines
Reaction Mechanism and Substrate Scope
The foundational method for synthesizing 5,10-dihydrophenazine involves the cyclocondensation of substituted catechols with o-phenylenediamines in high-boiling solvents such as ethylene glycol . This reaction proceeds via a stepwise mechanism:
-
Nucleophilic attack of the amine group on the catechol’s hydroxyl-bearing carbon.
-
Elimination of water to form a dihydroquinoxaline intermediate.
-
Aromatization through further dehydration, yielding the dihydrophenazine core .
Substituents on both reactants influence the reaction kinetics and product distribution. Electron-donating groups (e.g., methyl, methoxy) on the catechol accelerate the reaction, while bulky substituents on the diamine component may lead to steric hindrance . The patent literature demonstrates that this method accommodates diverse substrates, including 3-methylcatechol and 4-chloro-o-phenylenediamine, with isolated yields of 57% for 5,10-dimethyldihydrophenazine .
Optimization of Reaction Conditions
Critical parameters for maximizing yield include:
-
Solvent : Ethylene glycol preferred due to its high boiling point and ability to solubilize inorganic byproducts
-
Catalyst : Phase transfer agents (e.g., methyltributylammonium chloride) enhance alkylation efficiency in subsequent N-substitution steps
A representative procedure from US Patent 6,242,602B1 details:
10.0 g 3,3′-diaminobenzidine and 34.7 g 4-methylcatechol in 40 mL ethylene glycol heated at 212°C for 24 hours, followed by precipitation with water .
Reduction of Phenazine to this compound
Sodium Dithionite-Mediated Reduction
Phenazine undergoes facile reduction to this compound using sodium dithionite (Na₂S₂O₄) in aqueous ethanol . The reaction mechanism involves two-electron transfer, with the dithionite ion acting as a strong reducing agent:
This method achieves near-quantitative yields (98–99%) under optimized conditions . Key advantages include:
-
Ambient temperature operation (20–25°C)
-
Short reaction times (<2 hours)
N-Alkylation of Dihydrophenazine
The reduced product undergoes regioselective alkylation at the N5 and N10 positions using alkyl halides in the presence of phase transfer catalysts. A typical procedure employs:
-
Alkylating agent : Methyl iodide (3 equivalents)
-
Base : Sodium carbonate (2.5 equivalents)
-
Catalyst : Methyltributylammonium chloride (5 mol%)
Notably, maintaining a reducing environment with excess sodium dithionite prevents re-oxidation to phenazine during alkylation .
Palladium-Catalyzed Synthesis of 5,10-Diaryldihydrophenazines
Cross-Coupling Methodology
A groundbreaking advancement involves Pd(0)-mediated coupling of dihydrophenazine with aryl halides. The ACS Organic Letters report details:
-
Catalyst system : Pd₂(dba)₃ (2 mol%) with Xantphos ligand (4 mol%)
-
Base : Cs₂CO₃ (3 equivalents)
This method enables the introduction of diverse aryl groups (e.g., 4-methoxyphenyl, 2-naphthyl) with yields up to 85% . The reaction proceeds through oxidative addition of the aryl halide to Pd(0), followed by transmetallation and reductive elimination.
Substrate Compatibility Table
Aryl Halide | Dihydrophenazine Derivative | Yield (%) | Reference |
---|---|---|---|
4-Bromotoluene | 5,10-Di-p-tolyl | 78 | |
1-Iodonaphthalene | 5,10-Dinaphthyl | 82 | |
4-Bromoanisole | 5,10-Di(4-methoxyphenyl) | 85 |
Comparative Analysis of Synthetic Methods
Yield and Scalability Considerations
Method | Average Yield (%) | Scalability | Key Limitation |
---|---|---|---|
Condensation | 50–60 | Industrial | High-temperature requirements |
Reduction/Alkylation | 90–99 | Lab-scale | Requires pre-formed phenazine |
Pd-catalyzed Coupling | 70–85 | Research | Catalyst cost |
Oxidation Stability Challenges
All methods must address the propensity of this compound to oxidize to phenazine. Effective strategies include:
-
Sparging reactions with inert gases (N₂, Ar)
Recent Advances in Derivatization Techniques
Solid-Phase Alkylation
A patent-pending approach utilizes polymer-supported quaternary ammonium salts to facilitate N-alkylation in heterogeneous systems. This innovation reduces catalyst loading by 40% compared to traditional phase transfer methods .
Electrochemical Synthesis
Preliminary studies demonstrate the feasibility of generating this compound via cathodic reduction of phenazine at −1.2 V vs. Ag/AgCl. This method eliminates chemical reducing agents but currently suffers from low faradaic efficiency (32%) .
Industrial-Scale Production Insights
Purification Protocols
Large-scale manufacturing employs tandem purification steps:
-
Charcoal treatment : Removes colored impurities (e.g., 12 g charcoal per 500 mL toluene)
-
Solvent recrystallization : Toluene/ethanol mixtures optimize crystal morphology
-
Sublimation : For high-purity grades (>99.9%), vacuum sublimation at 150–180°C
Waste Management Considerations
The condensation method generates aqueous waste containing ethylene glycol and inorganic salts. Patent literature recommends:
-
Distillation recovery of ethylene glycol (85% efficiency)
-
Neutralization of alkaline wash streams with HCl before disposal
Emerging Applications Influencing Synthesis Design
The growing use of 5,10-dihydrophenazines in organic light-emitting diodes (OLEDs) has driven demand for:
-
High-purity materials : <100 ppm metal contaminants
-
Functionalized derivatives : 5,10-Bis(trifluoromethyl) variants for electron transport layers
These requirements necessitate modifications to traditional synthesis, such as implementing palladium scavengers in cross-coupling reactions .
Chemical Reactions Analysis
Types of Reactions
5,10-Dihydrophenazine undergoes various chemical reactions, including:
Oxidation: It can be oxidized to form phenazine derivatives.
Reduction: The compound can be reduced to form different dihydrophenazine derivatives.
Substitution: It can undergo substitution reactions to introduce various functional groups at the 5 and 10 positions.
Common Reagents and Conditions
Major Products Formed
Scientific Research Applications
Synthesis of 5,10-Dihydrophenazine Derivatives
The synthesis of this compound derivatives has been extensively studied due to their potential applications. Key synthetic methods include:
- Palladium-Catalyzed Reactions : The Buchwald–Hartwig reaction allows for the efficient synthesis of 5,10-diaryl-5,10-dihydrophenazines through arylation reactions. This method facilitates the introduction of diverse aryl groups onto the nitrogen atoms of the phenazine core .
- Iron-Catalyzed C–H Amination : Recent advancements have introduced iron-catalyzed intramolecular C–H amination techniques that yield 2,7-disubstituted derivatives with high regioselectivity. This method is notable for its ability to produce compounds with various substituents at the 2 and 7 positions of the phenazine core .
Applications in Organic Electronics
One of the most promising applications of this compound derivatives is in organic electronic devices:
- Hole-Injection Materials : These compounds have shown excellent properties as hole-injection materials in organic electroluminescent (OEL) devices. Their ability to facilitate charge transport makes them suitable for use in organic light-emitting diodes (OLEDs) and organic solar cells .
- Optoelectronic Properties : Theoretical studies using density functional theory (DFT) have revealed that these compounds exhibit favorable optoelectronic properties, enhancing their performance in electronic applications .
Medicinal Chemistry Applications
In addition to their electronic applications, this compound derivatives are being explored for their medicinal properties:
- Anticancer Activity : Synthetic derivatives of phenazine 5,10-dioxide have demonstrated potent cytotoxicity against human leukemic cells. These compounds exploit hypoxic environments typical in tumors to selectively induce cell death .
- Prodrug Strategies : The development of prodrugs based on this compound scaffolds has shown promise in enhancing bioactivity and solubility, making them potential candidates for cancer therapy .
Case Studies and Research Findings
Several studies highlight the efficacy and versatility of this compound derivatives:
Mechanism of Action
The mechanism of action of 5,10-dihydrophenazine involves multiple pathways:
Membrane Integrity: It affects the integrity of bacterial cell membranes, leading to increased susceptibility to oxidative stress.
Protein Synthesis: The compound interferes with amino acid and protein synthesis, disrupting bacterial growth and survival.
Virulence Modulation: It modulates the expression of virulence-related proteins, reducing the pathogenicity of bacteria.
Comparison with Similar Compounds
Phenazine 5,10-Dioxide
Structural and Functional Differences
Phenazine 5,10-dioxide derivatives are less redox-active but exhibit strong mutagenicity, limiting their therapeutic use . In contrast, dihydrophenazines are biosynthetic intermediates in prenylated antibiotics like endophenazines .
5,10-Diaryl-5,10-Dihydrophenazines
Substituent Effects on Performance
Symmetrical diaryl derivatives with electron-withdrawing groups (e.g., CF₃) enhance photocatalytic reducing power, while bulky substituents (e.g., t-Bu) improve stability . Asymmetric derivatives, such as 5-aryl-10-alkyl variants, are less explored but show promise in tailored catalysis .
Prenylated Dihydrophenazines
Data Tables
Table 1: Catalytic Performance of Dihydrophenazine Derivatives
Catalyst | Reaction | Yield (%) | Turnover Number (TON) | Reference |
---|---|---|---|---|
Phz2 (CF₃Ph/t-Bu) | Alkylation of silyl ether | 92 | 45 | |
PhenN-CF₃ | MMA polymerization | 65.9 | 1.5 × 10³ | |
PCBN | O-ATRP of acrylates | 90 | 2.0 × 10³ |
Table 2: Electrochemical Properties
Compound | E₀* (V vs SCE) | ΔEₛₜ (eV) | Lifetime (τ, µs) | |
---|---|---|---|---|
This compound core | −2.06 to −2.36 | 0.10–0.19 | 0.8–1.5 | |
Ir(ppy)₃ (reference) | −1.73 | 0.30 | 1.0 |
Biological Activity
5,10-Dihydrophenazine (H2Phen) is a compound of significant interest due to its diverse biological activities, particularly in the fields of oncology and microbiology. This article explores its biological activity, synthesis, and potential therapeutic applications based on recent research findings.
Overview of this compound
This compound is a derivative of phenazine, a nitrogen-containing heterocyclic compound known for its redox properties and ability to interact with biological systems. The compound's structure allows it to participate in various biochemical processes, making it a candidate for drug development.
Synthesis and Structural Modifications
The synthesis of this compound can be achieved through microbial transformation or chemical synthesis. Pseudomonas cepacia has been reported to convert phenazine into this compound under low oxygen tensions . Additionally, structural modifications can enhance its biological activity. For instance, derivatives with specific substituents have shown increased potency against cancer cells .
Anticancer Properties
Recent studies have highlighted the potent anticancer activity of this compound derivatives. Notably:
- Cytotoxicity Against Leukemia Cells : Compounds derived from this compound exhibit significant cytotoxic effects against acute myeloid leukemia (AML) cells. The most potent analogs demonstrate effective cell death at sub-micromolar concentrations . The mechanism involves the generation of reactive oxygen species (ROS), leading to oxidative stress and subsequent apoptosis in cancer cells .
- Selectivity : The selectivity of these compounds towards cancer cells over normal cells is a crucial feature. For example, EC50 values indicate that some derivatives are up to 40 times more effective against leukemia cells compared to non-cancerous cells .
Antimicrobial Activity
This compound also exhibits significant antimicrobial properties. It has been shown to inhibit the growth of various bacterial strains, making it a potential candidate for developing new antibiotics. The mechanism of action may involve DNA intercalation and inhibition of topoisomerases .
Table 1: Summary of Biological Activities
Activity Type | Target Cells/Organisms | EC50 Values (µM) | Mechanism of Action |
---|---|---|---|
Cytotoxicity | AML Cells (MOLM-13) | < 0.1 | ROS generation, apoptosis |
Antimicrobial | Various Bacterial Strains | Variable | DNA intercalation, topoisomerase inhibition |
Hypoxic Selectivity | Cancer Cells in Hypoxic Conditions | < 0.05 | Bioreductive activation |
Case Study: Iodinin and Myxin Analogues
Research on analogs such as iodinin and myxin has demonstrated their ability to induce cell death selectively in hypoxic environments typical of tumors. This hypoxia-selective mechanism enhances their therapeutic potential in cancer treatment .
Q & A
Basic Research Questions
Q. What synthetic routes are available for preparing 5,10-dihydrophenazine and its derivatives?
this compound derivatives are typically synthesized via oxidative coupling or functionalization of phenazine precursors. For example, 1,3-dihydroxyphenazine can be prepared through dithionite reduction of 1,3-dihydroxyphenazine dioxide, followed by purification via recrystallization in ethanol . Substituents such as methyl groups are introduced via alkylation reactions using reagents like methyl iodide under basic conditions . Characterization often involves nuclear magnetic resonance (NMR), high-performance liquid chromatography (HPLC), and elemental analysis to confirm purity and structure.
Q. How are spectroscopic techniques employed to characterize this compound?
Ultraviolet-visible (UV-Vis) spectroscopy reveals a λmax at 350 nm for this compound, distinct from phenazine (325 nm), due to differences in conjugation and electronic transitions . Infrared (IR) spectroscopy identifies N–H and C–N stretching vibrations in the 3200–3400 cm<sup>−1</sup> and 1350–1500 cm<sup>−1</sup> ranges, respectively. Diffuse reflectance spectroscopy further distinguishes solid-state optical properties, such as visible-region absorption in phenazhydrins .
Q. What are the key physicochemical properties of this compound?
Key properties include:
- Melting point : 153°C (for 5,10-dimethyl derivative) .
- Redox behavior : Exhibits reversible oxidation/reduction in electrochemical studies, critical for photoredox applications .
- Solubility : Generally soluble in polar aprotic solvents (e.g., DMF, THF) but insoluble in water .
Advanced Research Questions
Q. How do N,N-diaryl substituents influence charge transfer (CT) states in dihydrophenazine photoredox catalysts?
Substituents with extended conjugation (e.g., naphthyl groups) lower the LUMO energy, creating intramolecular CT states upon photoexcitation. This is confirmed by solvatochromic shifts in emission spectra (e.g., blue in hexane to red in DMF) and density functional theory (DFT) calculations . CT states enhance electron transfer efficiency in organic photocatalysis, such as in atom-transfer radical polymerization (O-ATRP), by minimizing radiative recombination .
Q. How is this compound utilized in thermally activated delayed fluorescence (TADF) materials?
As an electron donor in donor-acceptor (D-A) architectures, dihydrophenazine derivatives (e.g., DHPZ-2BI, DHPZ-2TRZ) achieve small singlet-triplet energy gaps (ΔEST ≈ 0–0.19 eV), enabling efficient reverse intersystem crossing (RISC). This property is exploited in organic light-emitting diodes (OLEDs) for green-to-orange emission, with external quantum efficiencies (EQE) exceeding 30% .
Q. What mechanistic insights explain the role of dihydrophenazine in photocatalytic CO2 reduction?
In CO2-to-CH4 conversion, dihydrophenazine photosensitizers (e.g., 5,10-di(2-naphthyl)-5,10-dihydrophenazine) absorb visible light (λ > 435 nm), transfer electrons to iron porphyrin catalysts, and mediate CO2 activation. Turnover numbers (TONs) reach 29 for CH4, with tertiary amines serving as sacrificial electron donors .
Q. How do solvent polarity and ion pairing affect dihydrophenazine-based O-ATRP performance?
Polar solvents stabilize ion pairs between the catalyst radical cation and bromide anion, crucial for deactivation of propagating polymer chains. Transient absorption spectroscopy reveals that solvent polarity modulates the lifetime of excited CT states, impacting polymerization control and dispersity (Đ < 1.2) .
Q. What enzymatic pathways involve dihydrophenazine derivatives in natural product biosynthesis?
The enzyme dihydrophenazine-1-carboxylate dimethylallyltransferase (PpzP) prenylates this compound-1-carboxylate using dimethylallyl diphosphate. This reaction is critical in Streptomyces anulatus for producing antimicrobial phenazines .
Q. Data Contradiction Analysis
Properties
IUPAC Name |
5,10-dihydrophenazine | |
---|---|---|
Source | PubChem | |
URL | https://pubchem.ncbi.nlm.nih.gov | |
Description | Data deposited in or computed by PubChem | |
InChI |
InChI=1S/C12H10N2/c1-2-6-10-9(5-1)13-11-7-3-4-8-12(11)14-10/h1-8,13-14H | |
Source | PubChem | |
URL | https://pubchem.ncbi.nlm.nih.gov | |
Description | Data deposited in or computed by PubChem | |
InChI Key |
IVURTNNWJAPOML-UHFFFAOYSA-N | |
Source | PubChem | |
URL | https://pubchem.ncbi.nlm.nih.gov | |
Description | Data deposited in or computed by PubChem | |
Canonical SMILES |
C1=CC=C2C(=C1)NC3=CC=CC=C3N2 | |
Source | PubChem | |
URL | https://pubchem.ncbi.nlm.nih.gov | |
Description | Data deposited in or computed by PubChem | |
Molecular Formula |
C12H10N2 | |
Source | PubChem | |
URL | https://pubchem.ncbi.nlm.nih.gov | |
Description | Data deposited in or computed by PubChem | |
DSSTOX Substance ID |
DTXSID80323047 | |
Record name | 5,10-dihydrophenazine | |
Source | EPA DSSTox | |
URL | https://comptox.epa.gov/dashboard/DTXSID80323047 | |
Description | DSSTox provides a high quality public chemistry resource for supporting improved predictive toxicology. | |
Molecular Weight |
182.22 g/mol | |
Source | PubChem | |
URL | https://pubchem.ncbi.nlm.nih.gov | |
Description | Data deposited in or computed by PubChem | |
CAS No. |
613-32-1 | |
Record name | 613-32-1 | |
Source | DTP/NCI | |
URL | https://dtp.cancer.gov/dtpstandard/servlet/dwindex?searchtype=NSC&outputformat=html&searchlist=402913 | |
Description | The NCI Development Therapeutics Program (DTP) provides services and resources to the academic and private-sector research communities worldwide to facilitate the discovery and development of new cancer therapeutic agents. | |
Explanation | Unless otherwise indicated, all text within NCI products is free of copyright and may be reused without our permission. Credit the National Cancer Institute as the source. | |
Record name | 5,10-dihydrophenazine | |
Source | EPA DSSTox | |
URL | https://comptox.epa.gov/dashboard/DTXSID80323047 | |
Description | DSSTox provides a high quality public chemistry resource for supporting improved predictive toxicology. | |
Synthesis routes and methods
Procedure details
Retrosynthesis Analysis
AI-Powered Synthesis Planning: Our tool employs the Template_relevance Pistachio, Template_relevance Bkms_metabolic, Template_relevance Pistachio_ringbreaker, Template_relevance Reaxys, Template_relevance Reaxys_biocatalysis model, leveraging a vast database of chemical reactions to predict feasible synthetic routes.
One-Step Synthesis Focus: Specifically designed for one-step synthesis, it provides concise and direct routes for your target compounds, streamlining the synthesis process.
Accurate Predictions: Utilizing the extensive PISTACHIO, BKMS_METABOLIC, PISTACHIO_RINGBREAKER, REAXYS, REAXYS_BIOCATALYSIS database, our tool offers high-accuracy predictions, reflecting the latest in chemical research and data.
Strategy Settings
Precursor scoring | Relevance Heuristic |
---|---|
Min. plausibility | 0.01 |
Model | Template_relevance |
Template Set | Pistachio/Bkms_metabolic/Pistachio_ringbreaker/Reaxys/Reaxys_biocatalysis |
Top-N result to add to graph | 6 |
Feasible Synthetic Routes
Disclaimer and Information on In-Vitro Research Products
Please be aware that all articles and product information presented on BenchChem are intended solely for informational purposes. The products available for purchase on BenchChem are specifically designed for in-vitro studies, which are conducted outside of living organisms. In-vitro studies, derived from the Latin term "in glass," involve experiments performed in controlled laboratory settings using cells or tissues. It is important to note that these products are not categorized as medicines or drugs, and they have not received approval from the FDA for the prevention, treatment, or cure of any medical condition, ailment, or disease. We must emphasize that any form of bodily introduction of these products into humans or animals is strictly prohibited by law. It is essential to adhere to these guidelines to ensure compliance with legal and ethical standards in research and experimentation.