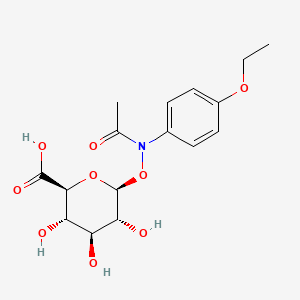
N-Hydroxyphenacetin glucuronide
- Click on QUICK INQUIRY to receive a quote from our team of experts.
- With the quality product at a COMPETITIVE price, you can focus more on your research.
Overview
Description
N-Hydroxyphenacetin glucuronide (NHPG) is a reactive metabolite of phenacetin, formed via N-hydroxylation followed by glucuronidation. It is a promutagen implicated in species-specific toxicity, particularly in hamsters, where its formation is significantly higher than in rats . NHPG has a half-life of ~8.7–9.0 hours in vitro and decomposes into phenacetin, acetaminophen, acetamide, 2-hydroxyphenacetin glucuronide, and protein-binding reactive intermediates (e.g., N-acetyl-p-benzoquinoneimine) . These intermediates are associated with mutagenicity and covalent protein adduction, contributing to organ toxicity .
Scientific Research Applications
Metabolic Pathways and Formation
N-Hydroxyphenacetin is primarily produced via the action of cytochrome P450 enzymes, specifically CYP1A2, which hydroxylates phenacetin. Subsequent conjugation reactions lead to the formation of N-hydroxyphenacetin glucuronide, which is then excreted in urine. Studies indicate that this compound can undergo further metabolism, highlighting its role in the biotransformation of phenacetin in various species, including humans and laboratory animals .
Toxicological Implications
Research has identified this compound as a potential promutagen, suggesting that it may contribute to the genotoxicity associated with phenacetin use. It has been shown to generate reactive metabolites that can interact with cellular macromolecules, leading to DNA damage. For instance, studies using hamster liver enzymes demonstrated that this metabolite could activate phenacetin into mutagenic forms .
Table 1: Toxicological Studies on this compound
Study Reference | Findings |
---|---|
IARC (1980) | Identified as a major metabolic route in multiple species. |
Health Council of the Netherlands (2012) | Linked to renal toxicity and potential carcinogenicity in long-term studies. |
Karger (2018) | Demonstrated instability with a half-life of 8.7 hours, leading to phenacetin reformation. |
Pharmacological Applications
While primarily studied for its toxicological properties, this compound also holds promise in pharmacology:
- Analgesic Activity : As a metabolite of phenacetin, it may retain some analgesic properties, contributing to the overall efficacy of phenacetin as a pain reliever.
- Antitumor Activity : Preliminary studies suggest that compounds related to N-hydroxyphenacetin exhibit cytotoxic effects against cancer cell lines, indicating potential applications in oncology .
Table 2: Antitumor Activity Studies
Cell Line | IC50 (µM) | Reference |
---|---|---|
MCF-7 | 0.045 | |
A549 | 0.038 | |
DU145 | 0.050 |
Case Studies
Several case studies have explored the implications of this compound in clinical settings:
- Cancer Treatment : In vitro studies demonstrated that this compound could induce apoptosis in breast cancer models while sparing normal cells, suggesting its potential as a targeted therapy.
- Infection Control : The compound has shown antimicrobial activity against multi-drug resistant bacterial strains, highlighting its versatility beyond analgesia and cancer treatment .
Q & A
Q. Basic: What analytical methods are recommended for quantifying N-Hydroxyphenacetin glucuronide in biological matrices?
Liquid chromatography-tandem mass spectrometry (LC-MS/MS) is the gold standard for quantifying glucuronide conjugates in biological samples. Key considerations include:
- Sample preparation : Use solid-phase extraction (SPE) with mixed-mode sorbents (e.g., Oasis HLB) to isolate the glucuronide from urine or plasma .
- Chromatography : Optimize reverse-phase columns (e.g., C18 or phenyl-hexyl) with gradient elution to separate this compound from matrix interferences .
- Mass detection : Employ negative-ion electrospray ionization (ESI) for glucuronides, monitoring specific transitions (e.g., precursor → aglycone fragment) .
- Validation : Include stability tests under storage conditions (-20°C for ≤4 months) and account for urinary creatinine normalization to mitigate variability .
Q. Advanced: How to design experiments to study metabolic activation pathways of this compound?
To investigate reactive intermediate formation:
- In vitro models : Use hepatic microsomes or recombinant UDP-glucuronosyltransferases (UGTs) to assess conjugation. Co-incubate with sulfotransferase inhibitors (e.g., pentachlorophenol) to isolate glucuronidation pathways .
- Covalent binding assays : Incubate radiolabeled this compound with albumin or glutathione (GSH) to trap reactive metabolites like N-acetyl-p-benzoquinoneimine. Quantify adducts via scintillation counting or LC-MS .
- Buffer selection : Avoid phosphate buffers, which can artifactually generate 3-hydroxyphenacetin phosphate. Use Tris buffer with ascorbic acid to stabilize intermediates .
Q. Basic: What are the key stability considerations for handling this compound in laboratory settings?
- Temperature : Store at -80°C in anhydrous DMSO or methanol to prevent hydrolysis. Avoid freeze-thaw cycles .
- pH : Decomposition accelerates at neutral or alkaline pH. Maintain acidic conditions (pH 4–5) during extraction .
- Half-life monitoring : Under physiological conditions (37°C, pH 7.4), the glucuronide has a half-life of ~9 hours. Include stabilizers like bovine serum albumin (BSA) to slow degradation .
Q. Advanced: How to resolve contradictory data on reactive metabolite formation from this compound?
Conflicting results often arise from competing decomposition pathways. Mitigate this by:
- Isotopic labeling : Synthesize deuterated or ¹⁴C-labeled glucuronide to track specific intermediates via high-resolution MS .
- Time-course studies : Profile metabolites at multiple timepoints (0–24 hrs) to distinguish primary (e.g., acetaminophen) vs. secondary (e.g., acetamide) products .
- Computational modeling : Use Bayesian analysis or Markov chain Monte Carlo (MCMC) simulations to quantify flux through competing pathways (e.g., hydrolysis vs. redox cycling) .
Q. Basic: What enzymatic systems are involved in the conjugation of N-Hydroxyphenacetin?
- UGT isoforms : Human UGT1A6 and UGT1A9 are primarily responsible for N-O-glucuronidation. Confirm activity using recombinant enzyme assays .
- Species differences : Rodent liver microsomes exhibit higher glucuronidation rates than humans, necessitating cross-species validation .
- Co-factor requirements : Supplement with uridine 5′-diphosphoglucuronic acid (UDPGA) in vitro to maintain enzymatic activity .
Q. Advanced: How to optimize synthesis protocols for this compound?
- Glycosylation strategies : Use protected glucuronic acid donors (e.g., trichloroacetimidates) and BF₃·OEt₂ catalysis for stereoselective coupling to N-Hydroxyphenacetin .
- Purification : Employ preparative HPLC with ion-pairing agents (e.g., tetrabutylammonium acetate) to resolve anomeric mixtures .
- Stability testing : Validate synthetic batches via accelerated degradation studies (40°C/75% RH) to ensure compliance with ICH guidelines .
Comparison with Similar Compounds
Comparative Analysis with Structurally Similar Glucuronides
N-Hydroxy-2-Acetylaminofluorene (N-Hydroxy-2-AAF) Glucuronide
- Stability and Reactivity: Unlike NHPG, N-hydroxy-2-AAF glucuronide is more stable and isolable. Its breakdown generates reactive intermediates, including N-hydroxy-2-aminofluorene glucuronide, which undergoes microsomal deacetylation to form DNA-binding species .
- Metabolic Activation : Both NHPG and N-hydroxy-2-AAF glucuronide decompose into electrophilic intermediates. However, NHPG’s decomposition is accelerated in phosphate buffer, forming 3-hydroxyphenacetin phosphate, a pathway absent in N-hydroxy-2-AAF .
Table 1: Stability and Reactivity of NHPG vs. N-Hydroxy-2-AAF Glucuronide
Acyl Glucuronides (e.g., Ibuprofen Acyl Glucuronide)
- Degradation Kinetics: Acyl glucuronides exhibit hydrolysis, anomerization, and transacylation. NHPG shares hydrolysis pathways but lacks transacylation due to its N-O-glucuronide linkage. Fast-reacting acyl glucuronides (e.g., unsubstituted phenylacetic acid derivatives) have half-lives <1 hour, while NHPG is slower (t.="" hours)="" li="" sub>="" ~9=""> Structural Influence: The carboxylate group in acyl glucuronides increases transacylation rates, whereas NHPG’s reactivity is driven by the N-O bond’s lability. Di-methylated acyl glucuronides (t1/2 23.3 hours) are slower than NHPG .
Table 2: Kinetic Comparison of NHPG with Acyl Glucuronides
Compound Type | t1/2 (hours) | Key Degradation Pathways |
---|---|---|
NHPG | 8.7–9.0 | Hydrolysis, rearrangement |
Unsubstituted Acyl Gluc. | <1 | Transacylation, hydrolysis |
Di-Methylated Acyl Gluc. | 23.3 | Hydrolysis |
Functional Comparison with Sulfated Metabolites
N-Hydroxyphenacetin Sulfate vs. NHPG
- Reactivity : The sulfate conjugate of N-hydroxyphenacetin is highly unstable (t1/2 <1 hour) and rearranges instantaneously into reactive metabolites, unlike the semi-stable NHPG .
- Mutagenicity : Both conjugates generate mutagenic intermediates, but NHPG’s slower degradation allows systemic distribution, contributing to urinary promutagenicity in hamsters .
Species-Specific Metabolism
- Hamsters vs. Rats : Hamster liver enzymes produce higher yields of N-hydroxyphenacetin, leading to greater NHPG formation and urinary mutagenicity compared to rats .
Structural and Metabolic Influences on Reactivity
- Linkage Type : NHPG is an O-glucuronide, whereas regorafenib-glucuronide (an N-glucuronide) is processed by distinct gut microbial enzymes, highlighting linkage-specific metabolic fates .
- Electronic Effects : The carboxylate group in acyl glucuronides enhances transacylation, absent in NHPG, where electronic effects on the N-O bond dominate degradation .
Preparation Methods
Enzymatic Synthesis of N-Hydroxyphenacetin Glucuronide
UDP-Glucuronosyltransferase (UGT)-Mediated Glucuronidation
The primary method for NHPG synthesis involves enzymatic glucuronidation of N-hydroxyphenacetin (NHP) using hepatic microsomes or recombinant UGT isoforms. Human liver microsomes incubated with NHP (100–500 μM), UDPGA (5 mM), and MgCl₂ (10 mM) in Tris-HCl buffer (pH 7.4) yield NHPG at rates of 0.8–1.2 nmol/min/mg protein . Recombinant UGT1A9 demonstrates the highest activity, with a kinetic efficiency (k<sub>cat</sub>/K<sub>m</sub>) of 12.4 mL/min/mg, compared to <5 mL/min/mg for UGT1A1 and UGT2B7 . Alamethicin (25 μg/mg protein) is essential to permeabilize microsomal membranes and overcome enzyme latency .
Key Reaction Conditions
Immobilized Enzyme Systems
Early studies utilized immobilized rabbit liver glucuronyl transferase bound to Sepharose 4B, achieving a 68% conversion efficiency of NHP to NHPG over 2 hours . This method, though less common today, provided foundational insights into enzyme kinetics and substrate specificity .
Chemical Synthesis and Stability Considerations
Direct Glycosylation Reactions
Chemical synthesis of NHPG via Koenigs-Knorr glycosylation has been attempted but yields (<15%) are inferior to enzymatic methods due to:
-
Steric hindrance from the N-acetyl group
-
Competitive oxidation of the N-hydroxylamine moiety
Stability Profile
NHPG exhibits pH-dependent hydrolysis:
pH | Half-life (37°C) | Major Degradation Product |
---|---|---|
5.0 | 42 min | N-Hydroxyphenacetin |
7.4 | 8 min | Phenacetin quinone imine |
9.0 | <2 min | Multiple undefined products |
Stabilization requires argon atmosphere and ascorbic acid (2 mM) to prevent oxidative degradation .
Metabolic Formation In Vivo
Hepatic Biotransformation Pathways
Phenacetin undergoes sequential metabolism to form NHPG:
-
CYP1A2-mediated N-deacetylation :
PhenacetinCYP1A2N-Hydroxyphenacetin
K<sub>m</sub> = 31 μM, V<sub>max</sub> = 4.1 nmol/min/mg -
UGT1A9-mediated glucuronidation :
N-HydroxyphenacetinUGT1A9NHPG
Hepatic extraction ratio = 0.92 (first-pass metabolism)
Interindividual Variability
UGT1A9 polymorphism (UGT1A9−275T>A/−2152C>T) reduces NHPG formation by 38–64% in poor metabolizers, increasing susceptibility to phenacetin toxicity .
Analytical Characterization
Chromatographic Methods
HPLC Conditions for NHPG Quantification :
Column | Mobile Phase | Flow Rate | Retention Time |
---|---|---|---|
C18 Aquasil | 10 mM NH₄OAc (pH 6.8)/ACN | 1 mL/min | 14.2 min |
Detection: UV at 338 nm (ε = 21,560 M⁻¹cm⁻¹) |
Thin-Layer Chromatography (TLC) :
-
Silica gel 60 F₂₅₄ plates
-
Solvent: Chloroform/methanol/ammonia (65:25:4 v/v)
-
R<sub>f</sub> = 0.37 (vs. 0.61 for NHP)
Spectroscopic Characterization
-
Glucuronide anomeric proton: δ 5.32 (d, J = 7.2 Hz)
-
N-Acetyl methyl: δ 2.12 (s)
-
Aromatic protons: δ 7.24–7.56 (m, 4H)
Electron Impact MS (Trimethylsilyl Derivative) :
-
m/z 742 [M]⁺ (calculated for C₂₄H₃₈N₂O₁₀Si₂: 742.2)
-
Key fragments: m/z 457 (aglycone + TMS), m/z 285 (glucuronic acid-TMS₂)
Challenges and Optimization Strategies
Low Yield in Chemical Synthesis
Strategies to improve yield include:
-
Microwave-assisted synthesis : 45% yield at 80°C (10 min vs. 24 h conventional)
-
Phase-transfer catalysis : Tetrabutylammonium bromide increases solubility in dichloromethane/water systems
Enzyme Inhibition
Co-administered drugs impact NHPG formation:
Inhibitor | IC₅₀ (μM) | Mechanism |
---|---|---|
Probenecid | 12.4 | UDPGA depletion |
Fluconazole | 89.7 | Competitive UGT binding |
Curcumin | 2.1 | Allosteric modulation |
Comparative Analysis of Preparation Methods
Method | Yield (%) | Purity (%) | Time (h) | Cost (USD/g) |
---|---|---|---|---|
Recombinant UGT1A9 | 78–92 | >99 | 2–4 | 1,200 |
Human liver microsomes | 45–68 | 95–98 | 6–8 | 850 |
Chemical synthesis | 12–18 | 88–92 | 24–48 | 2,400 |
Enzymatic methods using recombinant UGT1A9 provide the optimal balance of efficiency and scalability, though microsomal preparations remain valuable for metabolic studies .
Properties
CAS No. |
69783-19-3 |
---|---|
Molecular Formula |
C16H21NO9 |
Molecular Weight |
371.34 g/mol |
IUPAC Name |
(2S,3S,4S,5R,6S)-6-(N-acetyl-4-ethoxyanilino)oxy-3,4,5-trihydroxyoxane-2-carboxylic acid |
InChI |
InChI=1S/C16H21NO9/c1-3-24-10-6-4-9(5-7-10)17(8(2)18)26-16-13(21)11(19)12(20)14(25-16)15(22)23/h4-7,11-14,16,19-21H,3H2,1-2H3,(H,22,23)/t11-,12-,13+,14-,16-/m0/s1 |
InChI Key |
CGQCCRQISHOSGC-HAFSJTIUSA-N |
SMILES |
CCOC1=CC=C(C=C1)N(C(=O)C)OC2C(C(C(C(O2)C(=O)O)O)O)O |
Isomeric SMILES |
CCOC1=CC=C(C=C1)N(C(=O)C)O[C@H]2[C@@H]([C@H]([C@@H]([C@H](O2)C(=O)O)O)O)O |
Canonical SMILES |
CCOC1=CC=C(C=C1)N(C(=O)C)OC2C(C(C(C(O2)C(=O)O)O)O)O |
Synonyms |
N-hydroxyphenacetin glucuronide phenacetin N-O-glucuronide |
Origin of Product |
United States |
Disclaimer and Information on In-Vitro Research Products
Please be aware that all articles and product information presented on BenchChem are intended solely for informational purposes. The products available for purchase on BenchChem are specifically designed for in-vitro studies, which are conducted outside of living organisms. In-vitro studies, derived from the Latin term "in glass," involve experiments performed in controlled laboratory settings using cells or tissues. It is important to note that these products are not categorized as medicines or drugs, and they have not received approval from the FDA for the prevention, treatment, or cure of any medical condition, ailment, or disease. We must emphasize that any form of bodily introduction of these products into humans or animals is strictly prohibited by law. It is essential to adhere to these guidelines to ensure compliance with legal and ethical standards in research and experimentation.