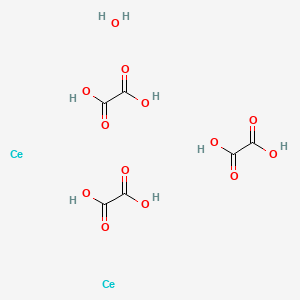
Cerium(III) oxalate
Overview
Description
Cerium(III) oxalate is a useful research compound. Its molecular formula is C6H2Ce2O13 and its molecular weight is 562.3. The purity is usually 95%.
BenchChem offers high-quality this compound suitable for many research applications. Different packaging options are available to accommodate customers' requirements. Please inquire for more information about this compound including the price, delivery time, and more detailed information at info@benchchem.com.
Mechanism of Action
Target of Action
Cerium(III) oxalate hydrate, also known as this compound, is an inorganic cerium salt of oxalic acid It is used as an antiemetic , suggesting that it may interact with receptors or enzymes involved in nausea and vomiting.
Mode of Action
It is known that cerium salts can increase the blood coagulation rate
Biochemical Pathways
Given its role in increasing the blood coagulation rate , it may be inferred that it affects the coagulation cascade, a complex series of reactions that lead to the formation of a clot.
Pharmacokinetics
It is known to be slightly soluble in water , which could impact its absorption and distribution
Result of Action
It is known that cerium salts can cause sensitivity to heat . Additionally, oxalates are corrosive to tissue and are powerful irritants. They have a caustic effect on the linings of the digestive tracts and can cause kidney damage .
Action Environment
Environmental factors can influence the action, efficacy, and stability of this compound hydrate. For instance, the presence of an oxidizing acid solution can facilitate the oxidation of Cerium(III) ions to Cerium(IV) ions . Additionally, this compound hydrate is known to convert to the oxide when heated , which could impact its stability and efficacy.
Biochemical Analysis
Biochemical Properties
Cerium(III) oxalate plays a role in biochemical reactions primarily through its interactions with biomolecules such as enzymes and proteins. One of the notable interactions is with oxalate oxidase, an enzyme that catalyzes the oxidation of oxalate to carbon dioxide and hydrogen peroxide. This compound can act as a substrate for this enzyme, leading to the production of reactive oxygen species (ROS) which can have various downstream effects on cellular processes .
Additionally, this compound interacts with proteins involved in oxidative stress responses. For instance, it can bind to antioxidant proteins such as superoxide dismutase (SOD) and catalase, potentially modulating their activity. These interactions can influence the cellular redox state and impact various signaling pathways .
Cellular Effects
This compound has been shown to affect various types of cells and cellular processes. In particular, it can influence cell function by altering cell signaling pathways, gene expression, and cellular metabolism. For example, exposure to this compound can lead to the activation of stress-responsive signaling pathways such as the mitogen-activated protein kinase (MAPK) pathway. This activation can result in changes in gene expression, including the upregulation of genes involved in oxidative stress responses and inflammation .
Furthermore, this compound can impact cellular metabolism by affecting the activity of key metabolic enzymes. It has been observed to inhibit enzymes involved in the tricarboxylic acid (TCA) cycle, leading to alterations in energy production and metabolic flux .
Molecular Mechanism
The molecular mechanism of action of this compound involves its interactions with biomolecules at the molecular level. This compound can bind to enzymes and proteins, leading to changes in their activity. For example, it can inhibit the activity of enzymes such as aconitase and succinate dehydrogenase in the TCA cycle, resulting in reduced energy production .
Additionally, this compound can induce the production of ROS through its interaction with oxalate oxidase. The generated ROS can cause oxidative damage to cellular components, including lipids, proteins, and DNA. This oxidative stress can trigger various cellular responses, including the activation of signaling pathways and changes in gene expression .
Temporal Effects in Laboratory Settings
In laboratory settings, the effects of this compound can change over time due to its stability and degradation. This compound is relatively stable under standard conditions, but it can undergo degradation when exposed to high temperatures or acidic environments . Over time, the degradation of this compound can lead to the formation of cerium oxide and other byproducts, which may have different biochemical properties and effects on cellular function .
Long-term exposure to this compound in in vitro and in vivo studies has shown that it can cause persistent oxidative stress and inflammation. These effects can result in chronic changes in cellular function, including alterations in cell proliferation, apoptosis, and differentiation .
Dosage Effects in Animal Models
The effects of this compound vary with different dosages in animal models. At low doses, this compound has been observed to have minimal toxic effects and can even exhibit protective effects against oxidative stress . At high doses, this compound can cause significant toxicity, including damage to the liver, kidneys, and other organs .
Threshold effects have been observed, where the toxic effects of this compound become apparent only above a certain dosage. For example, high doses of this compound can lead to severe oxidative damage, inflammation, and tissue necrosis .
Metabolic Pathways
This compound is involved in various metabolic pathways, particularly those related to oxidative stress and energy metabolism. It interacts with enzymes such as oxalate oxidase, which catalyzes the oxidation of oxalate to carbon dioxide and hydrogen peroxide . This reaction generates ROS, which can impact various metabolic processes .
Additionally, this compound can affect the TCA cycle by inhibiting key enzymes such as aconitase and succinate dehydrogenase. This inhibition can lead to alterations in metabolic flux and energy production, resulting in changes in cellular metabolism .
Transport and Distribution
This compound is transported and distributed within cells and tissues through various mechanisms. It can be taken up by cells via endocytosis and distributed to different cellular compartments . Within cells, this compound can interact with transporters and binding proteins that facilitate its movement and localization .
The distribution of this compound within tissues can also be influenced by its solubility and stability. In tissues, this compound can accumulate in specific regions, such as the liver and kidneys, where it can exert its biochemical effects .
Subcellular Localization
The subcellular localization of this compound can affect its activity and function. This compound can be localized to various cellular compartments, including the cytoplasm, mitochondria, and lysosomes . Its localization can be influenced by targeting signals and post-translational modifications that direct it to specific organelles .
In the mitochondria, this compound can interact with enzymes involved in the TCA cycle and oxidative phosphorylation, leading to changes in energy production and metabolic flux . In the lysosomes, this compound can induce oxidative stress and damage to cellular components .
Biological Activity
Cerium(III) oxalate (Ce(C₂O₄)₃) is a compound of cerium that has garnered attention for its biological activity, particularly in biomedical applications and environmental remediation. This article delves into its biological properties, mechanisms of action, and potential therapeutic uses, supported by relevant studies and data tables.
Overview of this compound
Cerium is a rare earth element known for its unique chemical properties. This compound is formed when cerium ions react with oxalic acid, resulting in a precipitate that can exist in various morphologies depending on the synthesis conditions. The biological activity of cerium compounds, particularly in the form of nanoparticles, has been extensively studied due to their antioxidant properties and potential applications in treating oxidative stress-related conditions.
The biological activity of this compound primarily involves its role as an antioxidant. Cerium nanoparticles (CNPs), which can include this compound, exhibit several mechanisms:
- Scavenging Free Radicals : CNPs can catalyze the decomposition of reactive oxygen species (ROS), reducing oxidative stress in cells. This is particularly relevant in renal epithelial cells exposed to high oxalate levels, where CNPs have been shown to restore mitochondrial function and reduce cell death .
- Inhibition of Crystal Formation : Studies indicate that cerium compounds can inhibit the formation of calcium oxalate crystals, which are implicated in kidney stone disease. By reducing the adhesion of these crystals to renal tubular cells, this compound may help prevent kidney damage .
Case Studies and Research Findings
-
Renal Protection Studies :
- A study demonstrated that cerium oxide nanoparticles effectively reduced oxidative stress and prevented calcium oxalate crystal deposition in rat kidneys. High-throughput sequencing revealed that these nanoparticles downregulated genes associated with cell adhesion, thus protecting renal epithelial cells from damage caused by hyperoxaluria .
- Catalytic Properties :
-
Nucleation and Growth Dynamics :
- In situ studies using liquid-cell transmission electron microscopy revealed insights into the nucleation and growth mechanisms of this compound nanocrystals. These findings are crucial for understanding how cerium compounds behave in biological systems and their potential applications in drug delivery or as therapeutic agents .
Data Table: Biological Effects of this compound
Scientific Research Applications
Catalytic Applications
Ozonation and Organic Pollutant Degradation
Cerium(III) oxalate has been utilized as a catalyst in the ozonation process for degrading organic pollutants. Recent studies have shown that cerium(III)-doped graphitic carbon nitride composites significantly enhance the mineralization of oxalate. The presence of cerium(III) increases the degradation efficiency by facilitating the formation of hydroxyl radicals, which are crucial for the oxidative breakdown of organic compounds .
Hydrogen Production via the Ceria Cycle
Cerium(III) oxide plays a critical role in the ceria cycle, a thermochemical process for hydrogen production. This process involves the endothermic dissolution of cerium dioxide followed by hydrolysis to cerium(III) oxide. The high reactivity of cerium(III) oxide with steam makes it a promising candidate for sustainable hydrogen production .
Material Science
Nanocrystal Formation and Growth Mechanisms
Recent research employing liquid-cell transmission electron microscopy has provided insights into the nucleation and growth mechanisms of this compound nanocrystals. The studies demonstrated that the growth dynamics are influenced by precursor concentration, revealing non-classical growth mechanisms such as monomer-by-monomer addition and particle aggregation. Understanding these mechanisms is essential for optimizing the synthesis of nanomaterials with desired properties .
Morphology-Controlled Precipitation
The precipitation of this compound can be controlled to produce specific morphologies, such as flower-like structures or compact particles. By manipulating reaction conditions and using crystallization modifiers like polyvinylpyrrolidone, researchers have achieved tailored nanostructures with high surface areas, which are beneficial for various applications including catalysis and drug delivery .
Environmental Remediation
Heavy Metal Ion Removal
this compound has shown potential in environmental applications, particularly in the removal of heavy metal ions from wastewater. Its ability to form stable complexes with various metal ions allows it to be used effectively in remediation processes. Studies have indicated that cerium-based compounds can enhance the adsorption capacities for metals like lead and cadmium, making them valuable in pollution control strategies .
Biomedical Applications
Historical Use as an Antiemetic
Historically, this compound was used as an antiemetic until it was replaced by more effective antihistamines. While its medical use has diminished, ongoing research into cerium compounds suggests potential future applications in drug delivery systems or as therapeutic agents due to their unique properties .
Summary Table: Applications of this compound
Application Area | Description |
---|---|
Catalysis | Enhances ozonation processes for organic pollutant degradation; involved in hydrogen production cycles. |
Material Science | Facilitates controlled growth of nanocrystals; enables synthesis of tailored nanostructures. |
Environmental Remediation | Effective in removing heavy metal ions from wastewater through complexation mechanisms. |
Biomedical Applications | Historical use as an antiemetic; potential future applications in drug delivery systems. |
Chemical Reactions Analysis
Precipitation Reaction
The synthesis of cerium(III) oxalate typically involves a precipitation reaction between cerium nitrate and oxalic acid:
This reaction highlights the stoichiometric relationship between the reactants, leading to the formation of this compound as a solid precipitate.
Thermal Decomposition
This compound can be thermally decomposed to produce cerium dioxide (), which is a valuable material in catalysis and electronics. The decomposition reaction can be represented as follows:
This reaction typically occurs at elevated temperatures, leading to the release of carbon dioxide and water vapor.
Research Findings on Reaction Mechanisms
Recent studies have utilized advanced techniques like liquid cell transmission electron microscopy (LC-TEM) to observe the growth dynamics of this compound nanoparticles in real time. Findings suggest that:
-
The initial growth phase is characterized by monomer-by-monomer addition, leading to nearly spherical shapes at lower concentrations.
-
As concentrations increase, rapid nucleation occurs, resulting in more complex structures through coalescence events .
These observations provide insights into optimizing synthesis conditions for desired particle characteristics.
Q & A
Basic Research Questions
Q. What are the standard synthesis methods for cerium(III) oxalate, and how do reaction conditions influence crystallinity?
- Methodological Answer : this compound is commonly synthesized via precipitation by reacting cerium salts (e.g., Ce(NO₃)₃) with oxalic acid under controlled pH (3–5) and temperature (25–60°C). Crystallinity is enhanced by slow addition of reagents and aging the precipitate for 12–24 hours . For advanced applications, microwave-assisted solvothermal methods reduce particle agglomeration and improve phase purity .
Q. How does the solubility of this compound vary in ionic solutions (e.g., nitrate, sulfate)?
- Methodological Answer : Solubility is highly dependent on counterions. In iron(III) nitrate solutions, solubility decreases due to competitive complexation, whereas in uranyl nitrate, it increases slightly (0.12 g/L at 25°C). Use ICP-MS or gravimetric analysis to quantify solubility, ensuring ionic strength is controlled .
Q. What characterization techniques are critical for verifying this compound purity and structure?
- Methodological Answer :
- XRD : Identifies crystalline phases and detects impurities (e.g., CeO₂ from premature decomposition) .
- TGA : Monitors dehydration (20–220°C, ~20% mass loss) and decomposition to CeO₂ (270–450°C, ~29% mass loss) .
- ICP-MS : Confirms trace metal impurities (<10 ppm for high-purity research-grade samples) .
Q. What are the safety protocols for handling this compound in laboratory settings?
- Methodological Answer : Store in airtight containers away from moisture to prevent hydrolysis. Use PPE (gloves, goggles) to avoid eye/skin irritation (H319 hazard). Neutralize spills with sodium bicarbonate and dispose via hazardous waste protocols .
Advanced Research Questions
Q. How do discrepancies between theoretical and experimental mass loss during thermal decomposition arise?
- Methodological Answer : Theoretical mass loss for Ce₂(C₂O₄)₃·8H₂O → 2CeO₂ is 49.84%, but experimental values often deviate due to:
- Residual moisture (TGA baseline drift below 100°C).
- Incomplete oxidation (Ce³⁺ → Ce⁴⁺) at <450°C, detectable via XPS .
Q. Why does cerium oxalate decomposition yield nanoparticles with mixed Ce(III)/Ce(IV) oxidation states?
- Methodological Answer : During calcination (400–600°C), surface oxygen vacancies form, stabilizing Ce³⁺. XPS analysis shows ~30% Ce³⁺ in 5 nm CeO₂ nanoparticles vs. <5% in bulk. Adjust calcination time/temperature to tune oxygen vacancy density .
Q. How do structural parameters of this compound complexes vary across synthesis routes?
- Methodological Answer : XRD reveals shorter Ce–O(oxalate) bonds (2.465 Å) in monomeric complexes vs. polymeric forms (2.515–2.62 Å). Use sterically hindered ligands (e.g., 2.2.2-cryptand) to isolate monomeric structures for precise reactivity studies .
Q. How can conflicting data on decomposition intermediates (e.g., Ce(CO₃)(OH) vs. CeO₂) be resolved?
Preparation Methods
Conventional Precipitation Methods
The precipitation of cerium(III) oxalate from aqueous solutions remains the most widely used synthesis route due to its scalability and cost-effectiveness. Three primary methodologies have been optimized for industrial and research applications.
Rapid Addition of Solid Oxalic Acid at Ambient Temperature
In this method, solid oxalic acid (H₂C₂O₄) is introduced directly into a cerium(III) nitrate (Ce(NO₃)₃·6H₂O) solution at room temperature. The rapid mixing induces instantaneous precipitation, yielding fine this compound particles. Studies demonstrate that this approach produces powders with a median particle size of 24.69 µm and bulk densities of 0.45–0.52 g/cm³ . The resulting oxalate (designated CeI ) exhibits sedimentation rates and particle size distributions comparable to thorium oxalate, making it suitable for mixed-oxide nuclear fuel precursors .
Critical Parameters :
Slow Addition of Oxalic Acid Solution at Elevated Temperature
A modified approach involves the gradual addition of oxalic acid solution (50°C) to cerium(III) nitrate under continuous stirring. This method (yielding CeII ) reduces particle agglomeration, producing narrower size distributions (median: 26.12 µm) compared to CeI . The slower reaction kinetics favor the growth of well-defined crystals, as evidenced by scanning electron microscopy (SEM) images showing hexagonal platelet morphologies .
Thermal Decomposition Profile :
-
Dehydration : 20–220°C (mass loss ~20%, corresponding to bound water removal) .
-
Oxalate decomposition : 270–450°C (mass loss ~29%, forming CeO₂) .
Use of Cerium(IV) Nitrate as a Precursor
Substituting cerium(III) nitrate with cerium(IV) nitrate (Ce(NO₃)₄) under similar conditions (50°C, slow oxalic acid addition) generates CeIII powders. Despite identical calcination protocols, CeIII-derived CeO₂ exhibits 15% higher tap densities (1.78 g/cm³) than CeI, attributed to differences in precursor oxidation states and nucleation rates . However, CeIII’s particle morphology diverges significantly from thorium oxalate, limiting its utility in mixed-oxide systems .
Advanced Synthesis Techniques
Microwave-Assisted Solvothermal Methods
Emerging techniques leverage microwave irradiation to accelerate reaction kinetics and improve phase purity. In one protocol, a cerium nitrate-oxalic acid mixture is heated to 150°C under microwave conditions (300 W, 10 min), yielding nanocrystalline this compound with reduced agglomeration. This method cuts synthesis time by 80% compared to conventional aging but requires precise control over microwave power to prevent premature decomposition.
Liquid Cell Transmission Electron Microscopy (LC-TEM)
Real-time LC-TEM studies reveal mechanistic insights into this compound nucleation and growth. At Ce³⁺ concentrations of 5–20 mM, initial nanoparticle formation occurs within 30 seconds, followed by oriented attachment into dendritic structures (Fig. 1) . Higher oxalate concentrations (15–60 mM) suppress branching, favoring isotropic growth .
Key Observations :
Thermal Decomposition and Calcination
Calcination conditions profoundly influence the surface area and sintering behavior of this compound-derived CeO₂.
Calcination Temperature (°C) | Bulk Density (g/cm³) | Tap Density (g/cm³) | Median Particle Size (µm) |
---|---|---|---|
400 | 0.58 | 0.82 | 24.69 |
700 | 0.94 | 1.32 | 27.15 |
900 | 1.21 | 1.65 | 29.46 |
Increasing calcination temperatures from 400°C to 900°C enhance densification but marginally affect particle size, with median diameters rising from 24.69 µm to 29.46 µm . X-ray diffraction (XRD) confirms complete conversion to fluorite-structured CeO₂ at 360°C, with crystallite sizes expanding from 8 nm to 22 nm as temperatures rise .
Characterization of this compound Powders
Particle Size Analysis :
-
Sedimentation method : CeI powders exhibit Stokes’ law-derived diameters of 20–50 µm, aligning with thorium oxalate .
-
Laser diffraction (Mastersizer) : 99% of CeO₂ particles post-calcination are <70 µm, with minimal submicron fines .
Thermogravimetric Analysis (TGA) :
Comparative Analysis of Synthesis Methods
Method | Median Particle Size (µm) | Bulk Density (g/cm³) | Compatibility with ThO₂ |
---|---|---|---|
CeI (rapid solid addition) | 24.69 | 0.45 | High |
CeII (slow solution) | 26.12 | 0.49 | Moderate |
CeIII (Ce⁴⁺ precursor) | 28.34 | 0.62 | Low |
CeI’s compatibility with thorium oxalate makes it preferable for nuclear applications, whereas CeIII’s higher density suits catalytic supports requiring robust mechanical properties.
Applications Influenced by Preparation Methods
-
Nuclear fuels : CeI-derived CeO₂-ThO₂ pellets achieve 95% theoretical density after sintering at 1600°C, meeting reactor-grade specifications .
-
Catalysis : Microwave-synthesized nanocrystalline CeO₂ exhibits 30% higher surface area (78 m²/g) than conventional samples, enhancing oxygen storage capacity.
Properties
IUPAC Name |
cerium;oxalic acid;hydrate | |
---|---|---|
Source | PubChem | |
URL | https://pubchem.ncbi.nlm.nih.gov | |
Description | Data deposited in or computed by PubChem | |
InChI |
InChI=1S/3C2H2O4.2Ce.H2O/c3*3-1(4)2(5)6;;;/h3*(H,3,4)(H,5,6);;;1H2 | |
Source | PubChem | |
URL | https://pubchem.ncbi.nlm.nih.gov | |
Description | Data deposited in or computed by PubChem | |
InChI Key |
OGGMXARXHWPYSA-UHFFFAOYSA-N | |
Source | PubChem | |
URL | https://pubchem.ncbi.nlm.nih.gov | |
Description | Data deposited in or computed by PubChem | |
Canonical SMILES |
C(=O)(C(=O)O)O.C(=O)(C(=O)O)O.C(=O)(C(=O)O)O.O.[Ce].[Ce] | |
Source | PubChem | |
URL | https://pubchem.ncbi.nlm.nih.gov | |
Description | Data deposited in or computed by PubChem | |
Molecular Formula |
C6H8Ce2O13 | |
Source | PubChem | |
URL | https://pubchem.ncbi.nlm.nih.gov | |
Description | Data deposited in or computed by PubChem | |
Molecular Weight |
568.35 g/mol | |
Source | PubChem | |
URL | https://pubchem.ncbi.nlm.nih.gov | |
Description | Data deposited in or computed by PubChem | |
Physical Description |
Hygroscopic powder; [Sigma-Aldrich MSDS] | |
Record name | Cerium(III) oxalate hydrate | |
Source | Haz-Map, Information on Hazardous Chemicals and Occupational Diseases | |
URL | https://haz-map.com/Agents/20415 | |
Description | Haz-Map® is an occupational health database designed for health and safety professionals and for consumers seeking information about the adverse effects of workplace exposures to chemical and biological agents. | |
Explanation | Copyright (c) 2022 Haz-Map(R). All rights reserved. Unless otherwise indicated, all materials from Haz-Map are copyrighted by Haz-Map(R). No part of these materials, either text or image may be used for any purpose other than for personal use. Therefore, reproduction, modification, storage in a retrieval system or retransmission, in any form or by any means, electronic, mechanical or otherwise, for reasons other than personal use, is strictly prohibited without prior written permission. | |
CAS No. |
15750-47-7 | |
Record name | Tris[oxalate(2-)]dicerium hydrate | |
Source | European Chemicals Agency (ECHA) | |
URL | https://echa.europa.eu/information-on-chemicals | |
Description | The European Chemicals Agency (ECHA) is an agency of the European Union which is the driving force among regulatory authorities in implementing the EU's groundbreaking chemicals legislation for the benefit of human health and the environment as well as for innovation and competitiveness. | |
Explanation | Use of the information, documents and data from the ECHA website is subject to the terms and conditions of this Legal Notice, and subject to other binding limitations provided for under applicable law, the information, documents and data made available on the ECHA website may be reproduced, distributed and/or used, totally or in part, for non-commercial purposes provided that ECHA is acknowledged as the source: "Source: European Chemicals Agency, http://echa.europa.eu/". Such acknowledgement must be included in each copy of the material. ECHA permits and encourages organisations and individuals to create links to the ECHA website under the following cumulative conditions: Links can only be made to webpages that provide a link to the Legal Notice page. | |
Disclaimer and Information on In-Vitro Research Products
Please be aware that all articles and product information presented on BenchChem are intended solely for informational purposes. The products available for purchase on BenchChem are specifically designed for in-vitro studies, which are conducted outside of living organisms. In-vitro studies, derived from the Latin term "in glass," involve experiments performed in controlled laboratory settings using cells or tissues. It is important to note that these products are not categorized as medicines or drugs, and they have not received approval from the FDA for the prevention, treatment, or cure of any medical condition, ailment, or disease. We must emphasize that any form of bodily introduction of these products into humans or animals is strictly prohibited by law. It is essential to adhere to these guidelines to ensure compliance with legal and ethical standards in research and experimentation.