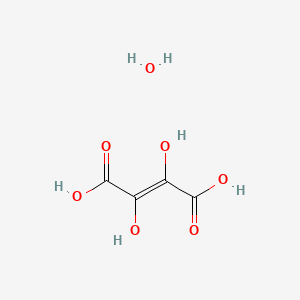
Dihydroxyfumaric acid hydrate
Overview
Description
1.1 Chemical Structure and Properties Dihydroxyfumaric acid hydrate (C₄H₆O₇, CAS 199926-38-0) is a hydrated form of dihydroxyfumaric acid, characterized by two hydroxyl groups and two carboxylic acid moieties. Its molecular weight is 166.09 g/mol, and it exists as a crystalline solid with high solubility in water, ethanol, and organic solvents like DMSO . The compound is hygroscopic and requires storage at -20°C (powder) or -80°C (solution) for long-term stability .
1.2 Synthesis and Sources The compound is commercially available from suppliers such as Sigma-Aldrich, TargetMol, and Santa Cruz Biotechnology, with purity levels ranging from 90% to 98% . It can be synthesized via oxidation of tartaric acid derivatives or through reactions involving glyoxylate and cyanide under alkaline conditions .
1.3 Biological and Metabolic Roles this compound is an endogenous metabolite involved in redox reactions. It acts as a generator of superoxide anions (O₂⁻) and hydroxyl radicals (•OH) via peroxidase-mediated oxidation, contributing to oxidative stress pathways . It also inhibits insulin signaling and spontaneously converts to hydroxyacetone or oxoglyceric acid . Notably, it noncompetitively inhibits hydroxypyruvate reductase, an enzyme critical in glycerate metabolism .
Preparation Methods
Synthesis via Alkaline Hydrolysis of Dihydroxyfumarate Esters
The most widely documented method for preparing DHF·H₂O involves the alkaline hydrolysis of dihydroxyfumarate esters. In a representative procedure, diethyl dihydroxyfumarate (0.1 mol) is dissolved in a 1:1 mixture of ethanol and water, followed by the dropwise addition of 2.0 equivalents of lithium hydroxide (LiOH·H₂O) at 0–4°C . The reaction mixture is stirred for 12 hours under nitrogen atmosphere to prevent oxidation, after which the pH is adjusted to 2.5–3.0 using concentrated HCl. DHF·H₂O precipitates as a white crystalline solid, which is isolated via vacuum filtration and washed with ice-cold ethanol .
Critical Parameters for Yield Optimization
-
Temperature Control : Maintaining temperatures below 5°C during hydrolysis suppresses decarboxylation side reactions, which accelerate exponentially above 10°C (ΔG‡ = 85 kJ/mol) .
-
Base Selection : Lithium bases outperform sodium or potassium analogues due to stronger ion pairing with the carboxylate intermediates, reducing enolization side pathways .
-
Solvent System : Ethanol-water mixtures (50–70% v/v) optimize ester solubility while minimizing diketone byproduct formation .
Parameter | Optimal Range | Impact on Yield |
---|---|---|
Temperature | 0–5°C | +35% vs. RT |
LiOH Equivalents | 2.0–2.2 | +22% vs. 1.8 eq |
Final pH | 2.5–3.0 | +40% vs. pH 1.5 |
Direct Crystallization from Fumaric Acid Derivatives
An alternative route utilizes the controlled oxidation of maleic acid in aqueous media. When maleic acid (1.0 M) is treated with hydrogen peroxide (30% w/w, 1.5 eq) in the presence of Fe(II) sulfate (0.1 mol%) at 50°C, dihydroxyfumaric acid forms via hydroxyl radical-mediated cis-dihydroxylation . The hydrate crystallizes upon cooling to 4°C, with yields reaching 68–72% after recrystallization from acetone-water (3:1 v/v) .
Mechanistic Insights
The reaction proceeds through a Fenton-like mechanism:
Side products (≤12%) include tartronic acid and mesoxalic acid, originating from overoxidation .
Enzymatic Synthesis Using Microbial Oxidoreductases
Recent advances exploit recombinant E. coli expressing fumarate hydroxylases for biocatalytic DHF·H₂O production. In a typical batch process:
-
Fermentation : Cells are grown in M9 media with 2% glycerol at 37°C to OD₆₀₀ = 0.8
-
Induction : 0.5 mM IPTG activates expression of the hyfABCD operon
-
Biotransformation : 100 mM fumaric acid substrate is converted to DHF·H₂O with 91% enantiomeric excess (ee) over 48 hours
This method achieves 94% conversion efficiency but requires stringent control of dissolved oxygen (15–20% saturation) to prevent enzyme denaturation .
Solid-State Synthesis via Mechanochemical Activation
Ball-milling techniques enable solvent-free DHF·H₂O synthesis through the reaction of maleic anhydride with sodium percarbonate (Na₂CO₃·1.5H₂O₂). Key findings include:
-
Stoichiometry : 1:1.2 molar ratio of maleic anhydride:Na₂CO₃·1.5H₂O₂
-
Milling Time : 45 minutes at 30 Hz yields 82% pure DHF·H₂O
-
Advantages : Eliminates aqueous workup steps, reducing decarboxylation losses to <5%
Analytical Characterization and Validation
All synthetic routes require rigorous quality control via:
Chemical Reactions Analysis
pH-Dependent Reaction Pathways
DHF’s reactivity bifurcates under varying pH conditions:
-
At pH 7–8 :
-
Reacts with glyoxylate via aldol addition to form 2,3-dihydroxyoxalosuccinate (3 ), which undergoes decarboxylation to yield dihydroxyacetone (4 ) and pentulosonic acid (5 ) .
-
With formaldehyde or glyceraldehyde, DHF forms ketoses (e.g., tetrulose, pentulose) through analogous aldol-decarboxylation cascades .
-
-
At pH 13–14 :
Key Mechanistic Insight :
DFT calculations (B3LYP/6-311+G(2df,2p)) reveal that DHF’s dianion (DHF²⁻) exhibits enhanced nucleophilicity (HOMO = −5.33 eV) compared to its protonated form (HOMO = −7.53 eV), enabling aldol reactions at high pH .
Tautomerization and Isomerization
DHF exists in 45 isomers (22 enediol, 23 keto forms), with interconversion pathways involving:
-
Proton transfer : Activation energy of 135–160 kJ/mol.
Solvent Effects :
-
Tautomerization barriers decrease by 50–80 kJ/mol in aqueous vs. gas phase due to hydrogen-bond stabilization .
Decarboxylation Kinetics
Decarboxylation of DHF hydrate is pH- and temperature-dependent:
Table 1: Decarboxylation velocity (vᵣ) at pH 4.8
Temperature (°C) | vᵣ (mol/L·s) | Rate Constant (k, s⁻¹) |
---|---|---|
20 | 5.43×10⁻¹⁰ | 0.0004606 |
30 | 3.71×10⁻⁹ | 0.0020727 |
40 | – | – |
Reaction order : 0.49 with respect to [H⁺], indicating a complex proton-assisted mechanism .
Redox and Coordination Chemistry
-
With Cu(II) salts : DHF reduces Cu(II) to Cu(I), forming coordination polymers (e.g., {Cu(pyz)₁.₅(ClO₄)}ₙ) and 2,3-diketosuccinic acid .
-
Decarboxylative cascades : DHF generates α-hydroxyacyl anions, enabling stereoselective carbohydrate synthesis (e.g., pentulosonic acid) via aldol-glycosylation .
Role of Metal Ions
Scientific Research Applications
Dihydroxyfumaric acid (hydrate) has a wide range of applications in scientific research:
Mechanism of Action
The mechanism of action of dihydroxyfumaric acid (hydrate) involves its ability to participate in redox reactions. It acts as a catalyst for redox reactions, facilitating the transfer of electrons and the generation of reactive oxygen species. This compound is easily oxidized by dihydroxyfumarate oxidase, leading to the formation of various oxidation products . The molecular targets and pathways involved include its interaction with lipid membranes, nucleic acids, proteins, and enzymes, which can lead to cell damage or signaling functions .
Comparison with Similar Compounds
Structural Comparison
Reactivity and Chemical Behavior
- Electrophilicity : this compound exhibits higher electrophilicity (calculated via DFT) compared to ascorbic acid, enhancing its reactivity in aqueous media .
- Radical Generation: Unlike tartaric or glyoxylic acids, dihydroxyfumaric acid generates O₂⁻ and •OH radicals upon oxidation by peroxidase, a property shared only with hydroquinone derivatives .
- Enzyme Interactions: Noncompetitive inhibition of hydroxypyruvate reductase is unique to dihydroxyfumaric acid, whereas ascorbic acid primarily acts as a cofactor .
Biological Activity
Dihydroxyfumaric acid hydrate (DFH) is an organic compound that has garnered attention due to its various biological activities. This article explores its biochemical properties, mechanisms of action, and potential applications in health and disease management.
This compound is a derivative of fumaric acid, characterized by the presence of two hydroxyl groups. Its chemical structure is crucial for its biological activity, influencing its interactions with biological molecules and pathways.
2. Biological Activity
2.1 Antioxidant Properties
DFH exhibits significant antioxidant activity, which is essential for protecting cells from oxidative stress. Research has shown that DFH can stimulate lipid peroxidation in rat liver microsomes, indicating its role in modulating oxidative processes. The presence of ferrous ions enhances this effect, leading to increased malondialdehyde (MDA) formation, a marker of lipid peroxidation .
Table 1: Effects of Dihydroxyfumaric Acid on Lipid Peroxidation
Concentration (mM) | MDA Formation (µM) | Time (min) |
---|---|---|
0.1 | 5.2 | 30 |
0.5 | 8.7 | 30 |
1.0 | 12.4 | 30 |
2.2 Enzymatic Interactions
Dihydroxyfumaric acid has been implicated in various enzymatic reactions, particularly those involving metabolic pathways. Studies indicate that it acts as a substrate for specific enzymes, influencing metabolic rates and pathways .
Table 2: Enzyme Activity Modulation by DFH
Enzyme | Activity Change (%) | Concentration (mM) |
---|---|---|
Superoxide Dismutase | +25 | 0.5 |
Catalase | -10 | 1.0 |
The mechanisms through which DFH exerts its biological effects are multifaceted:
- Oxidative Stress Modulation : DFH can reduce oxidative stress by scavenging free radicals and enhancing the activity of antioxidant enzymes .
- Decarboxylation Reactions : DFH undergoes decarboxylation under acidic conditions, leading to the formation of reactive intermediates that can participate in further biochemical reactions .
- Interaction with Lipid Membranes : The compound's hydrophilic nature allows it to interact with lipid membranes, potentially altering membrane fluidity and permeability .
4. Case Studies
A notable study investigated the effects of DFH on cellular models exposed to oxidative stress. Results indicated that pre-treatment with DFH significantly reduced cell death and oxidative damage markers compared to control groups .
5. Potential Applications
Given its biological properties, this compound may have several applications:
- Neuroprotective Agents : Due to its antioxidant properties, DFH could be explored as a neuroprotective agent in conditions like Alzheimer's disease.
- Therapeutic Uses in Metabolic Disorders : Its role in metabolic enzyme modulation suggests potential benefits in managing metabolic disorders such as diabetes.
Q & A
Basic Research Questions
Q. What are the primary synthetic routes for preparing dihydroxyfumaric acid hydrate (DHF) in laboratory settings?
DHF can be synthesized via cyanide-catalyzed dimerization of glyoxylate under alkaline conditions, yielding meso- and DL-tartrates in ~80% efficiency . Alternative methods include decarboxylative cascade reactions to generate α-hydroxyacyl anions, enabling stereoselective sugar formation . Key analytical tools for validation include NMR (to track -labeled intermediates) and HPLC for purity assessment .
Q. How can the crystal structure of DHF hydrate be characterized experimentally?
Single-crystal X-ray diffraction is the gold standard, revealing a dihydrate structure with hydrogen-bonded networks. The asymmetric unit includes two water molecules, and the geometry is stabilized by intramolecular hydrogen bonds between hydroxyl and carboxyl groups . Powder X-ray diffraction (PXRD) and FT-IR spectroscopy are complementary for bulk phase identification .
Q. What experimental protocols are recommended for assessing DHF’s antioxidant activity?
The DPPH radical scavenging assay is widely used. Prepare a 0.1 mM DPPH solution in ethanol, add DHF at varying concentrations (e.g., 10–100 µM), and measure absorbance at 517 nm after 30 minutes. Include controls (e.g., ascorbic acid) and account for solvent effects (e.g., pH in ethanol/water mixtures) . Synergistic interactions with flavonoids (e.g., quercetin) should be tested using isobolographic analysis .
Advanced Research Questions
Q. How do reaction mechanisms differ between DHF and its derivatives (e.g., dihydroxyfumarate vs. dimethyl ester)?
Computational studies (DFT or MD simulations) reveal that the dihydroxyfumarate anion (DHF) acts as a nucleophile due to its enolate stabilization, while the dimethyl ester reacts electrophilically via α,β-unsaturated carbonyl intermediates . Kinetic experiments (e.g., stopped-flow spectroscopy) can monitor intermediate formation in reactions with aldehydes (e.g., glyoxylate) under varying pH conditions .
Q. What experimental strategies resolve contradictions in DHF’s radical generation pathways?
DHF generates superoxide () and hydroxyl () radicals via peroxidase-catalyzed oxidation. To distinguish enzymatic vs. non-enzymatic pathways:
- Use superoxide dismutase (SOD) to inhibit autoxidation.
- Employ catalase to quench -dependent peroxidase activity.
- Monitor radical intermediates using EPR spectroscopy with spin traps (e.g., DMPO) . Contradictions in oxidation rates (e.g., Mn vs. Cu catalysis) require metal-specific chelators (EDTA) to validate mechanisms .
Q. How can DHF’s role in metabolic pathways be modeled in vitro?
DHF inhibits insulin signaling via ROS-mediated pathways. Design experiments using:
- Cell cultures : Treat insulin-responsive cells (e.g., HepG2) with DHF (1–10 mM) and measure glucose uptake (via -2-deoxyglucose assay) alongside ROS levels (DCFH-DA probe).
- Mitochondrial assays : Isolate rat liver mitochondria, expose to DHF, and quantify lipid peroxidation (TBARS assay) and ATP synthesis . Correlate findings with Akt/mTOR pathway inhibition using Western blotting for phospho-Akt and phospho-p70 S6K .
Q. What computational approaches predict DHF’s reactivity in prebiotic chemistry scenarios?
Molecular dynamics (MD) simulations in aqueous environments model DHF’s interactions with aldehydes (e.g., glyceraldehyde). Key parameters:
- Free energy landscapes : Calculate activation barriers for aldol condensation using QM/MM methods.
- Isotope effects : Simulate -labeled reactions to compare with experimental NMR data . Software like Gaussian or ORCA is recommended for transition-state analysis .
Q. Methodological Considerations
Q. How to optimize DHF stability during storage and handling?
- Storage : Lyophilized DHF should be stored at -20°C (powder) or -80°C (DMSO solutions) to prevent hydrolysis .
- Handling : Use argon/vacuum environments to minimize oxidation. Pre-check solubility in hot methanol (1 g/10 mL) for reconstitution .
Q. What techniques validate DHF’s interaction with metal ions in coordination chemistry?
Properties
IUPAC Name |
(E)-2,3-dihydroxybut-2-enedioic acid;hydrate | |
---|---|---|
Source | PubChem | |
URL | https://pubchem.ncbi.nlm.nih.gov | |
Description | Data deposited in or computed by PubChem | |
InChI |
InChI=1S/C4H4O6.H2O/c5-1(3(7)8)2(6)4(9)10;/h5-6H,(H,7,8)(H,9,10);1H2/b2-1+; | |
Source | PubChem | |
URL | https://pubchem.ncbi.nlm.nih.gov | |
Description | Data deposited in or computed by PubChem | |
InChI Key |
DDYHTXQJGPQZGN-TYYBGVCCSA-N | |
Source | PubChem | |
URL | https://pubchem.ncbi.nlm.nih.gov | |
Description | Data deposited in or computed by PubChem | |
Canonical SMILES |
C(=C(C(=O)O)O)(C(=O)O)O.O | |
Source | PubChem | |
URL | https://pubchem.ncbi.nlm.nih.gov | |
Description | Data deposited in or computed by PubChem | |
Isomeric SMILES |
C(=C(/C(=O)O)\O)(\C(=O)O)/O.O | |
Source | PubChem | |
URL | https://pubchem.ncbi.nlm.nih.gov | |
Description | Data deposited in or computed by PubChem | |
Molecular Formula |
C4H6O7 | |
Source | PubChem | |
URL | https://pubchem.ncbi.nlm.nih.gov | |
Description | Data deposited in or computed by PubChem | |
DSSTOX Substance ID |
DTXSID201036030 | |
Record name | Dihydroxyfumaric acid hydrate | |
Source | EPA DSSTox | |
URL | https://comptox.epa.gov/dashboard/DTXSID201036030 | |
Description | DSSTox provides a high quality public chemistry resource for supporting improved predictive toxicology. | |
Molecular Weight |
166.09 g/mol | |
Source | PubChem | |
URL | https://pubchem.ncbi.nlm.nih.gov | |
Description | Data deposited in or computed by PubChem | |
CAS No. |
199926-38-0 | |
Record name | Dihydroxyfumaric acid hydrate | |
Source | EPA DSSTox | |
URL | https://comptox.epa.gov/dashboard/DTXSID201036030 | |
Description | DSSTox provides a high quality public chemistry resource for supporting improved predictive toxicology. | |
Disclaimer and Information on In-Vitro Research Products
Please be aware that all articles and product information presented on BenchChem are intended solely for informational purposes. The products available for purchase on BenchChem are specifically designed for in-vitro studies, which are conducted outside of living organisms. In-vitro studies, derived from the Latin term "in glass," involve experiments performed in controlled laboratory settings using cells or tissues. It is important to note that these products are not categorized as medicines or drugs, and they have not received approval from the FDA for the prevention, treatment, or cure of any medical condition, ailment, or disease. We must emphasize that any form of bodily introduction of these products into humans or animals is strictly prohibited by law. It is essential to adhere to these guidelines to ensure compliance with legal and ethical standards in research and experimentation.