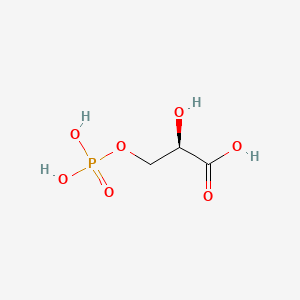
3-Phosphoglyceric acid
Overview
Description
3-Phosphoglyceric acid (3-PGA) is a 3-carbon intermediate metabolite with the molecular formula C₃H₇O₇P and an average molecular mass of 186.056 Da . It is a central molecule in both glycolysis and the Calvin cycle, where it serves as a precursor for ATP synthesis and carbon fixation, respectively . Structurally, 3-PGA features a phosphate group esterified to the third carbon of the glycerol backbone, distinguishing it from other phosphorylated glyceric acids (e.g., 2-phosphoglyceric acid) .
In glycolysis, 3-PGA is generated from 1,3-bisphosphoglyceric acid and subsequently converted to 2-phosphoglyceric acid by phosphoglycerate mutase (PGAM) . In the Calvin cycle, it is the first stable product of CO₂ fixation, enabling carbohydrate biosynthesis . Beyond these roles, 3-PGA is implicated in cancer metabolism, microbial energy regulation, and neurological health, as discussed in recent studies .
Preparation Methods
Synthetic Routes and Reaction Conditions: 3-Phosphoglyceric acid can be synthesized through the enzymatic dephosphorylation of 1,3-bisphosphoglycerate. This reaction is catalyzed by the enzyme phosphoglycerate kinase, which transfers a phosphate group from 1,3-bisphosphoglycerate to adenosine diphosphate, forming adenosine triphosphate and this compound .
Industrial Production Methods: Industrial production of this compound typically involves the use of microbial fermentation processes. Microorganisms such as Escherichia coli can be genetically engineered to overproduce enzymes involved in glycolysis, thereby increasing the yield of this compound .
Types of Reactions:
Oxidation and Reduction: this compound can undergo oxidation to form 3-phosphohydroxy pyruvate, catalyzed by the enzyme 3-phosphoglycerate dehydrogenase.
Substitution: The phosphate group in this compound can be substituted by other functional groups under specific conditions.
Common Reagents and Conditions:
Oxidation: Common reagents include nicotinamide adenine dinucleotide (NAD+) as an oxidizing agent.
Substitution: Various nucleophiles can be used to substitute the phosphate group, depending on the desired product.
Major Products:
Oxidation: 3-Phosphohydroxy pyruvate.
Substitution: Products vary based on the nucleophile used.
Scientific Research Applications
Metabolic Pathway Studies
3-Phosphoglyceric acid serves as a key intermediate in both glycolysis and the Calvin-Benson cycle:
- Glycolysis : In this pathway, this compound is produced from 1,3-bisphosphoglycerate through the action of the enzyme phosphoglycerate kinase. This reaction also generates ATP via substrate-level phosphorylation .
- Calvin-Benson Cycle : In photosynthesis, this compound is formed from the fixation of carbon dioxide by ribulose bisphosphate (RuBP) and is essential for synthesizing sugars .
Plant Physiology Research
Research has shown that this compound is integral to understanding plant metabolism:
- C3 and C4 Plants : Studies have demonstrated that the concentration of this compound varies significantly between C3 and C4 plant species, influencing their photosynthetic efficiency . For instance, it constitutes a significant portion of primary products in these plants, impacting growth and yield.
- Amino Acid Synthesis : It acts as a precursor for the synthesis of amino acids such as serine, which can further lead to the production of cysteine and glycine . This relationship is vital for understanding plant protein synthesis and metabolism.
Cancer Research
The role of this compound in cancer metabolism is gaining attention:
- Warburg Effect : This compound is implicated in the Warburg effect, where cancer cells preferentially utilize glycolysis for energy production even in the presence of oxygen. This metabolic shift allows for rapid cell proliferation and survival under hypoxic conditions .
Biochemical Assays
This compound is utilized in various biochemical assays:
- Chromatographic Techniques : It can be separated and quantified using techniques like paper chromatography and mass spectrometry. These methods are essential for studying its concentration in biological samples .
- Enzyme Activity Studies : The compound is used to investigate enzyme kinetics, particularly those involved in glycolysis and photosynthesis, providing insights into metabolic regulation .
Case Study 1: Photosynthetic Efficiency in C4 Plants
A study examined the role of this compound in enhancing photosynthetic efficiency among C4 plants. Researchers found that higher levels of this compound correlated with improved carbon fixation rates and biomass accumulation compared to C3 plants. The findings underscore the importance of metabolic intermediates in optimizing plant growth under varying environmental conditions.
Case Study 2: Targeting Cancer Metabolism
Another significant study focused on targeting the metabolic pathways involving this compound to inhibit cancer cell growth. By disrupting serine biosynthesis pathways linked to this compound, researchers were able to reduce proliferation rates in several cancer cell lines. This case highlights the potential for developing novel therapeutic strategies aimed at metabolic vulnerabilities in cancer cells.
Mechanism of Action
3-Phosphoglyceric acid exerts its effects primarily through its role as a metabolic intermediate. In glycolysis, it is involved in substrate-level phosphorylation, where it is converted to 2-phosphoglycerate by the enzyme phosphoglycerate mutase . In the Calvin-Benson cycle, it is formed from the fixation of carbon dioxide and is subsequently converted to glyceraldehyde 3-phosphate . The molecular targets include enzymes such as phosphoglycerate kinase and phosphoglycerate mutase .
Comparison with Similar Compounds
Structural and Functional Comparison
Table 1: Key Characteristics of 3-Phosphoglyceric Acid and Related Metabolites
Key Differences :
- Phosphate Position: 3-PGA and 2-phosphoglyceric acid are structural isomers, differing in phosphate group placement. This distinction determines their enzymatic processing—3-PGA is metabolized by PGAM, while 2-phosphoglyceric acid is converted to PEP by enolase .
- Biosynthetic Roles : 3-PGA is a hub for serine biosynthesis via phosphoglycerate dehydrogenase (PHGDH), a pathway upregulated in cancers .
Research Findings on Metabolic Roles and Dysregulation
Cancer Metabolism
- Elevated 3-PGA in Tumors: Plasma levels of 3-PGA are significantly higher in non-small cell lung cancer (NSCLC) patients compared to healthy controls, correlating with altered glycolysis and amino acid metabolism .
- PHGDH Inhibition: Natural compounds like salvianolic acids bind to PHGDH, blocking 3-PGA conversion to serine and suppressing tumor growth (MolDock scores: −189.11 Å for salvianolic acid C) .
Microbial Energy Regulation
- CR-hvKP Pathogens : Protocatechuic acid (PCA) inhibits PGAM in CR-hvKP, causing 3-PGA accumulation. This disrupts the pentose phosphate pathway (PPP), reducing NADPH and nucleic acid synthesis .
- Compensatory Mechanisms : Microbes upregulate bisphosphoglycerate mutase (BPGM) to mitigate 3-PGA toxicity, but PCA suppresses this adaptation .
Metabolic Recovery in Disease
- Pancreatic Damage : Mesenchymal stem cells (MSCs) reverse 3-PGA depletion in damaged pancreases, restoring glycolytic flux and reducing citric acid accumulation .
Neurological Implications
- However, gender-specific metabolic responses (e.g., aspartic acid elevation in females) complicate direct correlations .
Enzyme Interactions and Inhibitors
Chemical Reactions Analysis
Substrate-Level Phosphorylation
Phosphoglycerate kinase (PGK) catalyzes the reversible transfer of a phosphate group from 1,3-bisphosphoglycerate (1,3-BPG) to ADP, yielding ATP and 3-PGA:
This reaction is critical for ATP production during glycolysis. Structural studies reveal an SN2-type nucleophilic attack by ADP’s β-phosphate on 1,3-BPG’s 1-phosphate, with magnesium ions stabilizing the transition state .
Enzyme | Gene | UniProt ID | Molecular Weight |
---|---|---|---|
Phosphoglycerate kinase 1 | PGK1 | P00558 | 44,614 Da |
Phosphoglycerate kinase 2 | PGK2 | P07205 | 44,796 Da |
Phosphate Relocation
Phosphoglycerate mutase (PGAM) isomerizes 3-PGA to 2-phosphoglycerate (2-PGA) via a 2,3-bisphosphoglycerate (2,3-BPG) intermediate:
This reaction involves a phosphoenzyme intermediate where a histidine residue transiently binds the phosphate group. PGAM’s catalytic efficiency depends on Mn²⁺ coordination in the active site .
Enzyme | Gene | UniProt ID | Molecular Weight |
---|---|---|---|
Phosphoglycerate mutase 1 | PGAM1 | P18669 | 28,804 Da |
Phosphoglycerate mutase 2 | PGAM2 | P15259 | 28,766 Da |
Carbon Fixation and Cleavage
Rubisco catalyzes CO₂ fixation into ribulose-1,5-bisphosphate (RuBP), forming an unstable 6-carbon intermediate that spontaneously cleaves into two 3-PGA molecules:
This reaction is the primary carbon assimilation step in photosynthesis .
Phosphorylation for Reductive Phase
Phosphoglycerate kinase (PGK) phosphorylates 3-PGA to 1,3-BPG using ATP, enabling subsequent reduction to glyceraldehyde-3-phosphate (G3P):
This step is the reverse of the glycolytic PGK reaction .
Bisphosphoglycerate Metabolism
Bisphosphoglycerate mutase (BPGM) regulates hemoglobin oxygen affinity by converting 1,3-BPG to 2,3-BPG, which hydrolyzes to 3-PGA:
This pathway is critical for erythrocyte function .
Acylphosphatase Activity
Acylphosphatase-1/2 hydrolyzes 1,3-BPG directly to 3-PGA:
These enzymes exhibit secondary phosphatase activity but lack a well-defined physiological role .
Kinetic and Structural Insights
-
PHGDH Inhibition : Phenolic compounds like chicoric acid selectively inhibit PHGDH in cancer cells by binding its substrate site (Ki = 0.8 μM) .
-
PGAM Mechanism : Mn²⁺ ions in Bacillus stearothermophilus PGAM coordinate active-site histidines (His8, His59, His181), facilitating phosphate transfer .
-
ATP Coupling : PGK’s conformational changes align 1,3-BPG and ADP for in-line phosphoryl transfer, optimizing catalytic efficiency .
Q & A
Basic Research Questions
Q. What is the role of 3-phosphoglyceric acid in the Calvin cycle, and how can its metabolic flux be experimentally quantified?
3-PGA is a critical intermediate in the Calvin cycle, formed during CO₂ fixation by ribulose-1,5-bisphosphate carboxylase/oxygenase (RuBisCO). Methodologically, researchers employ isotopic labeling (e.g., ¹³C or ¹⁴C) combined with gas chromatography-mass spectrometry (GC-MS) to track carbon assimilation rates. For example, GC-EI-(TOF)MS enables precise identification of 3-PGA via its pertrimethylsilylated derivative (exact mass: 474.1511) . Flux balance analysis (FBA) can also model its distribution between sugar synthesis and ribulose-1,5-bisphosphate regeneration .
Q. How does 3-PGA contribute to glycolysis, and what experimental approaches validate its enzymatic conversions?
In glycolysis, 3-PGA is produced via oxidation of glyceraldehyde-3-phosphate and is subsequently converted to 2-phosphoglycerate. Researchers use coupled enzyme assays (e.g., monitoring NADH oxidation) and kinetic studies with purified enzymes like phosphoglycerate kinase (PGK) to quantify reaction rates. High-performance liquid chromatography (HPLC) or nuclear magnetic resonance (NMR) can track 3-PGA levels in cell lysates .
Q. What analytical techniques are recommended for quantifying 3-PGA in biological samples?
GC-MS with electron impact ionization (EI) is a gold standard for 3-PGA quantification due to its high sensitivity and specificity. The pertrimethylsilylated derivative of 3-PGA (C15H39O7PSi4) produces a distinct fragmentation pattern, enabling unambiguous identification . Liquid chromatography-tandem mass spectrometry (LC-MS/MS) is also widely used for metabolomic profiling in complex matrices .
Advanced Research Questions
Q. How can conflicting data on 3-PGA’s regulatory effects in the pentose phosphate pathway (PPP) be resolved?
3-PGA accumulation (e.g., due to PGAM inhibition) disrupts PPP by reducing NADPH and ribose-5-phosphate production. To resolve contradictions, researchers combine metabolomics (e.g., LC-MS-based quantification of PPP intermediates) with enzyme activity assays (e.g., glucose-6-phosphate dehydrogenase, G6PDH). Molecular docking studies further validate interactions between 3-PGA and PPP enzymes . For instance, hydrogen bonding between 3-PGA and G6PDH residues can be modeled to assess inhibition mechanisms .
Q. What experimental designs address 3-PGA’s dual role in energy metabolism (e.g., glycolysis vs. photorespiration)?
Controlled perturbation experiments, such as CRISPR-mediated knockout of PGAM or overexpression of bypass enzymes (e.g., bisphosphoglycerate mutase, BPGM), can isolate 3-PGA’s effects. Isotope tracer studies (e.g., ¹³C-glucose) combined with metabolic flux analysis (MFA) differentiate contributions to glycolysis and photorespiration. For example, ³¹P-NMR can monitor ATP/ADP ratios under varying 3-PGA concentrations .
Q. How do computational tools like Metaboverse identify 3-PGA-related regulatory networks in metabolomic datasets?
Metaboverse employs pattern recognition algorithms to rank reactions involving 3-PGA (e.g., its interconversion with glyceric acid) based on regulatory signatures. Researchers apply causal network analysis to prioritize metabolites linked to mitochondrial dysfunction or oxidative stress. For example, 3-PGA ranked 56th in causal networks in lung adenocarcinoma studies, highlighting its role in energy metabolism dysregulation .
Q. What methodologies elucidate 3-PGA’s allosteric regulation of enzymes like ADP-glucose pyrophosphorylase (AGPase)?
Site-directed mutagenesis of AGPase residues (e.g., Lys-197, Pro-261) combined with kinetic assays (Km and Vmax measurements) reveals 3-PGA’s activation mechanisms. Differential scanning fluorimetry (DSF) or surface plasmon resonance (SPR) can quantify binding affinities. Studies on potato AGPase mutants showed increased sensitivity to 3-PGA activation, confirming its role in starch biosynthesis .
Q. How can researchers reconcile discrepancies in 3-PGA’s ATP yield across different organisms or tissues?
Comparative studies using tissue-specific knockout models (e.g., liver vs. muscle cells) and thermodynamic modeling (e.g., Gibbs free energy calculations) account for variations in ATP yield. For example, 3-PGA’s contribution to ATP synthesis in C3 plants (via photorespiration) differs from its role in mammalian glycolysis. Stoichiometric models of the EMP pathway can adjust for NADPH/ATP ratios .
Q. What strategies validate 3-PGA as a biomarker in metabolic disorders?
Multi-cohort metabolomic studies with standardized protocols (e.g., ISO 15197) are essential. Machine learning algorithms (e.g., random forest classifiers) analyze 3-PGA levels in clinical datasets to distinguish pathologies like mitochondrial diseases. Longitudinal studies tracking 3-PGA in alveolar macrophages or serum samples correlate its fluctuations with disease progression .
Q. How do researchers design experiments to study 3-PGA’s epigenetic effects in immune cells?
Chromatin immunoprecipitation sequencing (ChIP-seq) and RNA-seq are used to assess 3-PGA’s impact on histone acetylation (e.g., H3K27ac) in macrophages. Pharmacological inhibition of 3-PGA synthesis (e.g., using PGAM inhibitors) followed by ATAC-seq identifies accessible chromatin regions. These findings link 3-PGA to metabolic reprogramming in innate immunity .
Q. Key Methodological Considerations
- Data Contradictions : Use orthogonal techniques (e.g., enzyme assays + molecular docking) to validate regulatory roles .
- Advanced Tools : Metaboverse for network analysis , GC-MS for quantification , and CRISPR for pathway perturbation .
- Experimental Design : Include controls for ATP/NADPH levels and isotope dilution effects in tracer studies .
Properties
IUPAC Name |
(2R)-2-hydroxy-3-phosphonooxypropanoic acid | |
---|---|---|
Details | Computed by Lexichem TK 2.7.0 (PubChem release 2021.10.14) | |
Source | PubChem | |
URL | https://pubchem.ncbi.nlm.nih.gov | |
Description | Data deposited in or computed by PubChem | |
InChI |
InChI=1S/C3H7O7P/c4-2(3(5)6)1-10-11(7,8)9/h2,4H,1H2,(H,5,6)(H2,7,8,9)/t2-/m1/s1 | |
Details | Computed by InChI 1.0.6 (PubChem release 2021.10.14) | |
Source | PubChem | |
URL | https://pubchem.ncbi.nlm.nih.gov | |
Description | Data deposited in or computed by PubChem | |
InChI Key |
OSJPPGNTCRNQQC-UWTATZPHSA-N | |
Details | Computed by InChI 1.0.6 (PubChem release 2021.10.14) | |
Source | PubChem | |
URL | https://pubchem.ncbi.nlm.nih.gov | |
Description | Data deposited in or computed by PubChem | |
Canonical SMILES |
C(C(C(=O)O)O)OP(=O)(O)O | |
Details | Computed by OEChem 2.3.0 (PubChem release 2021.10.14) | |
Source | PubChem | |
URL | https://pubchem.ncbi.nlm.nih.gov | |
Description | Data deposited in or computed by PubChem | |
Isomeric SMILES |
C([C@H](C(=O)O)O)OP(=O)(O)O | |
Details | Computed by OEChem 2.3.0 (PubChem release 2021.10.14) | |
Source | PubChem | |
URL | https://pubchem.ncbi.nlm.nih.gov | |
Description | Data deposited in or computed by PubChem | |
Molecular Formula |
C3H7O7P | |
Details | Computed by PubChem 2.2 (PubChem release 2021.10.14) | |
Source | PubChem | |
URL | https://pubchem.ncbi.nlm.nih.gov | |
Description | Data deposited in or computed by PubChem | |
DSSTOX Substance ID |
DTXSID40862427 | |
Record name | D-(-)-3-Phosphoglyceric acid | |
Source | EPA DSSTox | |
URL | https://comptox.epa.gov/dashboard/DTXSID40862427 | |
Description | DSSTox provides a high quality public chemistry resource for supporting improved predictive toxicology. | |
Molecular Weight |
186.06 g/mol | |
Details | Computed by PubChem 2.2 (PubChem release 2021.10.14) | |
Source | PubChem | |
URL | https://pubchem.ncbi.nlm.nih.gov | |
Description | Data deposited in or computed by PubChem | |
CAS No. |
820-11-1, 3443-58-1 | |
Record name | 3-phospho-D-glyceric acid | |
Source | DrugBank | |
URL | https://www.drugbank.ca/drugs/DB04510 | |
Description | The DrugBank database is a unique bioinformatics and cheminformatics resource that combines detailed drug (i.e. chemical, pharmacological and pharmaceutical) data with comprehensive drug target (i.e. sequence, structure, and pathway) information. | |
Explanation | Creative Common's Attribution-NonCommercial 4.0 International License (http://creativecommons.org/licenses/by-nc/4.0/legalcode) | |
Record name | D-(-)-3-Phosphoglyceric acid | |
Source | EPA DSSTox | |
URL | https://comptox.epa.gov/dashboard/DTXSID40862427 | |
Description | DSSTox provides a high quality public chemistry resource for supporting improved predictive toxicology. | |
Record name | D-Glycerate 3-phosphate | |
Source | Human Metabolome Database (HMDB) | |
URL | http://www.hmdb.ca/metabolites/HMDB0060180 | |
Description | The Human Metabolome Database (HMDB) is a freely available electronic database containing detailed information about small molecule metabolites found in the human body. | |
Explanation | HMDB is offered to the public as a freely available resource. Use and re-distribution of the data, in whole or in part, for commercial purposes requires explicit permission of the authors and explicit acknowledgment of the source material (HMDB) and the original publication (see the HMDB citing page). We ask that users who download significant portions of the database cite the HMDB paper in any resulting publications. | |
Disclaimer and Information on In-Vitro Research Products
Please be aware that all articles and product information presented on BenchChem are intended solely for informational purposes. The products available for purchase on BenchChem are specifically designed for in-vitro studies, which are conducted outside of living organisms. In-vitro studies, derived from the Latin term "in glass," involve experiments performed in controlled laboratory settings using cells or tissues. It is important to note that these products are not categorized as medicines or drugs, and they have not received approval from the FDA for the prevention, treatment, or cure of any medical condition, ailment, or disease. We must emphasize that any form of bodily introduction of these products into humans or animals is strictly prohibited by law. It is essential to adhere to these guidelines to ensure compliance with legal and ethical standards in research and experimentation.