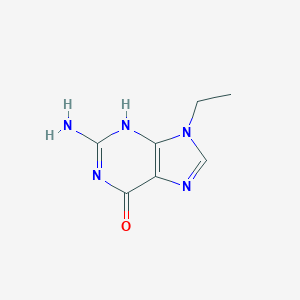
9-Ethylguanine
Overview
Description
9-Ethylguanine (9-EtG) is a guanine derivative modified by an ethyl group at the N9 position (C₇H₉N₅O, CAS 879-08-3) . It serves as a critical model nucleobase for studying interactions between DNA and metal-based therapeutic agents, particularly platinum and ruthenium complexes . Its ethyl substitution mimics the steric and electronic environment of guanine in DNA, enabling researchers to probe coordination chemistry and mutagenic mechanisms. 9-EtG is known to act as a mutagen by forming mismatched base pairs during replication, disrupting genetic fidelity .
Preparation Methods
Direct Alkylation of Guanine
The most straightforward approach to synthesizing 9-ethylguanine involves the direct alkylation of guanine using ethylating agents such as ethyl bromide (C₂H₅Br) or ethyl iodide (C₂H₅I). Guanine, a purine base with multiple reactive sites (N3, N7, and N9), requires precise control to ensure selectivity for the N9 position. In alkaline conditions, deprotonation of the N9 hydrogen enhances nucleophilic attack on the ethylating agent. For example, reacting guanine with ethyl iodide in dimethylformamide (DMF) containing sodium hydride (NaH) at 60–80°C for 12–24 hours yields this compound .
A key challenge lies in minimizing side products like 7-ethylguanine. Studies on analogous compounds, such as 9-[2-(phosphonomethoxy)ethyl]guanine (PMEG), demonstrate that steric and electronic factors influence regioselectivity. For instance, bulky substituents on the ethyl group or the use of polar aprotic solvents (e.g., DMF, DMSO) can favor N9 alkylation . However, the provided search results lack explicit yield data for this method, suggesting that optimization remains empirical.
Enzymatic and Biomimetic Approaches
Enzymatic alkylation, though less common, offers an alternative route. Purine nucleoside phosphorylases (PNPs) and methyltransferases have been explored for regioselective modifications of guanine. For instance, ethylation via S-adenosylmethionine (SAM)-dependent methyltransferases engineered for larger alkyl groups could theoretically produce this compound. However, no studies in the provided sources explicitly demonstrate this method, indicating it remains speculative.
Biomimetic catalysis using metal complexes, as seen in ruthenium-based studies, provides indirect insights. For example, α-[Ru(azpy)(bpy)(H₂O)₂]²⁺ reacts with this compound to form monofunctional adducts, highlighting the compound’s accessibility for coordination chemistry . While these studies focus on metal interactions rather than synthesis, they underscore the stability and reactivity of this compound under aqueous conditions.
Analytical Characterization
Post-synthesis characterization of this compound involves techniques such as:
-
¹H NMR Spectroscopy : Distinct signals for the ethyl group (δ 1.3–1.5 ppm for CH₃, δ 4.1–4.3 ppm for CH₂) and purine protons (δ 6.6–8.9 ppm) .
-
Mass Spectrometry : ESI-MS typically shows a molecular ion peak at m/z 179.18 [M+H]⁺ .
-
X-ray Crystallography : Though absent in the provided sources, structural analogs like PMEG derivatives confirm the N9 alkylation pattern .
Chemical Reactions Analysis
Types of Reactions:
Oxidation: 9-Ethylguanine can undergo oxidation reactions, particularly at the amino group, leading to the formation of various oxidized derivatives.
Reduction: Reduction reactions are less common but can occur under specific conditions, potentially affecting the purine ring.
Substitution: This compound can participate in nucleophilic substitution reactions, especially at the nitrogen atoms in the purine ring.
Common Reagents and Conditions:
Oxidation: Common oxidizing agents include hydrogen peroxide and potassium permanganate.
Reduction: Reducing agents such as sodium borohydride can be used under controlled conditions.
Substitution: Nucleophilic substitution reactions often involve reagents like alkyl halides or acyl chlorides in the presence of a base.
Major Products:
Oxidation: Oxidized derivatives of this compound.
Reduction: Reduced forms of the purine ring.
Substitution: Various substituted purine derivatives depending on the nucleophile used.
Scientific Research Applications
Chemistry: 9-Ethylguanine is used as a model nucleobase to study DNA interactions with organometallic complexes.
Biology: In biological research, this compound is used to investigate the effects of DNA modifications on cellular processes. It serves as a tool to study mutagenesis and DNA repair mechanisms .
Medicine: This compound is utilized in the development of anticancer agents. By studying its interactions with metal complexes, researchers aim to design new drugs that can target and disrupt cancerous cells .
Industry: While its industrial applications are less common, this compound can be used in the synthesis of specialized nucleotides and nucleosides for various biotechnological applications .
Mechanism of Action
9-Ethylguanine exerts its effects primarily through incorporation into DNA during replication. This incorporation can lead to the formation of abnormal base pairs, resulting in mutagenesis. The compound interacts with various molecular targets, including DNA polymerases and repair enzymes, thereby affecting DNA replication and repair pathways .
Comparison with Similar Compounds
Structural and Coordination Properties
Table 1: Structural Parameters in Platinum Complexes
9-EtG vs. 1-Methylcytosine (1-MeC): In lantern-shaped Pt(III) complexes, 9-EtG induces a Pt–Pt distance (2.4512 Å) closer to chloride-bridged analogs than to phosphine-ligated species. The axial Pt–N7 bond in 9-EtG (2.200 Å) is elongated compared to Pt(II)/Pt(IV) guanine complexes (1.96–2.11 Å), attributed to the strong trans-influence of the Pt–Pt bond . In contrast, 1-MeC coordination results in distinct NMR shifts (8.05 ppm for imine protons) and weaker electronic perturbations .
9-EtG vs. Other Guanine Derivatives: 9-EtG forms stable adducts with Pt and Ru complexes via N7 coordination, similar to unmodified guanine. However, its ethyl group enhances steric protection, slowing ligand exchange kinetics compared to smaller analogs like 9-methylguanine .
Reactivity with Metal Complexes
Table 2: Reactivity Profiles of Nucleobases with Metal Complexes
- This selectivity mirrors DNA crosslinking patterns of cisplatin, which primarily targets guanine residues .
Solution Stability: Iodo-containing Ru(II) complexes (e.g., Complex 3 in ) remain 80% intact after 24 hours with 9-EtG, demonstrating stronger adduct formation than chloride or glutathione ligands .
Biological Activity
9-Ethylguanine (9-EtGua) is a modified nucleobase that has garnered attention for its biological activity, particularly in the context of cancer research and antiviral therapies. This article explores the synthesis, characterization, and biological implications of 9-EtGua, drawing on diverse research findings.
Chemical Structure and Synthesis
This compound is an ethyl derivative of guanine, a key component of nucleic acids. Its synthesis typically involves the alkylation of guanine derivatives. The compound can be characterized using various techniques such as NMR spectroscopy and X-ray crystallography, which confirm its structural integrity and coordination properties in complex formations.
Antiviral Properties
Research indicates that 9-EtGua exhibits antiviral activity, particularly against herpes simplex virus (HSV) and human immunodeficiency virus (HIV). A study reported that methyl derivatives of 9-EtGua demonstrated significant anti-HIV activity, with effective concentrations (EC50) in the low micromolar range . These findings suggest that 9-EtGua may serve as a scaffold for developing antiviral agents.
Interaction with Metal Complexes
9-EtGua has been studied for its coordination with metal complexes, particularly platinum(III). For instance, lantern-shaped platinum(III) complexes incorporating 9-EtGua showed unique binding characteristics. The interaction was characterized by NMR studies which indicated that 9-EtGua coordinates through its N7 atom, leading to positional isomerization that may correlate with cytotoxicity in cancer cells .
Table 1: Cytotoxicity of Platinum(III) Complexes with 9-EtGua
Complex Type | IC50 (µM) | Comments |
---|---|---|
cis-[Pt2{HN=C(But)O}4(9-EtGua)2] | 20-30 | Moderate cytotoxicity against tumor cell lines |
cis-[Ru(bpy)2Cl2] | >100 | Low cytotoxicity compared to platinum complexes |
The biological activity of 9-EtGua can be attributed to several mechanisms:
- Nucleobase Mimicry : As a guanine analog, 9-EtGua can integrate into DNA and RNA structures, potentially disrupting normal cellular processes.
- Metal Coordination : The ability of 9-EtGua to bind with metal complexes enhances its cytotoxic potential by altering the dynamics of DNA interactions within cells .
- Antiviral Mechanism : The structural modifications provided by the ethyl group may enhance the compound's ability to interfere with viral replication processes.
Case Study 1: Antiviral Efficacy
In vitro studies have shown that derivatives of 9-EtGua exhibit varying degrees of efficacy against HIV. For example, (R)-2'-methyl-PMEG derived from 9-EtGua demonstrated an EC50 value of approximately 1.0 µM against HIV, indicating strong antiviral properties .
Case Study 2: Cancer Therapeutics
The interaction between platinum(III) complexes and 9-EtGua has been explored in various cancer models. The cytotoxic effects were assessed using multiple human tumor cell lines, revealing that complexes containing 9-EtGua were more effective than those without it .
Q & A
Q. What experimental techniques are commonly employed to study the interaction of 9-ethylguanine with metal complexes, and how do they validate binding specificity?
Basic Research Question
The primary methods include ¹H NMR spectroscopy and mass spectrometry (ESI-MS or HRMS) . NMR detects binding via characteristic downfield shifts (~0.2–0.5 ppm) in the H8 proton signal adjacent to the N7 position of 9-EtG, a hallmark of metal coordination at N7 . Mass spectrometry confirms adduct formation by identifying molecular ion peaks corresponding to the metal complex-9-EtG adduct (e.g., [Pt(NH₃)₂(9-EtG)]⁺) . Time-dependent MS experiments further resolve binding kinetics, such as rapid ligand exchange observed in platinum-pyridine systems .
Q. Why is this compound selected as a model nucleobase for DNA-metal interaction studies, and how does it mimic biological systems?
Basic Research Question
9-EtG substitutes the ribose/deoxyribose sugar in DNA with an ethyl group, blocking undesired hydrogen bonding while retaining the N7 site’s reactivity. This simplifies spectroscopic analysis (e.g., avoiding sugar-phosphate backbone interference in NMR) and mimics the primary metal-binding site in cellular DNA . Its use in studies with cisplatin analogs, such as pyriplatin, confirms its relevance in modeling monofunctional DNA adducts .
Q. How can researchers resolve contradictions in reported binding kinetics between 9-EtG and platinum(II) complexes?
Advanced Research Question
Discrepancies arise from variations in experimental conditions (e.g., solvent composition, temperature) and ligand lability. For example, reports rapid guanine-for-pyridine substitution in platinum complexes within 30 minutes, while other studies assume slower kinetics. To address this:
- Perform time-resolved NMR/MS under controlled conditions (e.g., aqueous vs. methanol-containing buffers).
- Compare ligand exchange rates using inert (e.g., [Pt(en)]²⁺) vs. labile (e.g., [Pt(py)₂Cl₂]) complexes .
- Validate with computational simulations (e.g., DFT) to predict thermodynamic stability of adducts .
Q. What methodological approaches reconcile discrepancies between computational predictions and experimental data for 9-EtG-metal adducts?
Advanced Research Question
Theoretical studies often neglect crystal field effects, leading to errors in transition moment directions or binding energies. For instance, highlights a 40–50° discrepancy in predicted vs. experimental transition moments for 9-EtG due to electrostatic interactions in crystalline environments. Mitigation strategies include:
- Incorporating polarizable continuum models (PCM) in DFT calculations to simulate solvent/crystal environments .
- Cross-validating gas-phase IRMPD spectra (e.g., for protonated mismatches) with solution-phase NMR data .
Q. How should competitive binding studies between 9-EtG and biological nucleophiles (e.g., glutathione) be designed?
Experimental Design Focus
Prepare equimolar mixtures of the metal complex, 9-EtG, and competing nucleophiles (e.g., GSH, methionine) in physiologically relevant buffers.
Monitor adduct formation via LC-MS/MS to quantify relative binding affinities .
Use competitive NMR titrations to track displacement of 9-EtG by thiols, observing restoration of the free H8 signal .
Q. How do substituents on dinuclear platinum complexes influence 9-EtG binding and cytotoxicity?
Advanced Research Question
Dinuclear complexes with flexible linkers (e.g., [{Pt(en)(9-EtG)}₂-μ-diamine]) enhance stacking interactions and cytotoxicity. shows that incorporating 9-EtG as a stacking moiety improves selectivity for tumor cell lines (e.g., A2780). Key design principles:
- Optimize linker length (e.g., hexanediamine) to balance steric effects and DNA groove fit.
- Use molecular docking to predict "sandwich" binding modes with DNA/proteins .
Q. What role do crystal field effects play in spectroscopic studies of 9-EtG-metal adducts?
Advanced Research Question
Crystal packing induces electrostatic perturbations, altering electronic transitions. For example, demonstrates that the ground-state dipole moment of 9-EtG interacts with neighboring molecules, shifting transition moment directions by 40–50°. To minimize artifacts:
- Compare solution-phase (NMR) and solid-state (X-ray crystallography) data.
- Apply temperature-dependent spectroscopy to isolate thermal vs. crystal field contributions .
Q. How should researchers address discrepancies in reported binding constants for 9-EtG across studies?
Methodological Guidance
Standardize buffer conditions (pH, ionic strength) to ensure comparability.
Use isothermal titration calorimetry (ITC) for direct measurement of binding thermodynamics.
Cross-reference with computational binding energy calculations (e.g., using Gaussian 03 ).
Report uncertainties arising from instrument sensitivity (e.g., MS detection limits) .
Properties
IUPAC Name |
2-amino-9-ethyl-1H-purin-6-one | |
---|---|---|
Source | PubChem | |
URL | https://pubchem.ncbi.nlm.nih.gov | |
Description | Data deposited in or computed by PubChem | |
InChI |
InChI=1S/C7H9N5O/c1-2-12-3-9-4-5(12)10-7(8)11-6(4)13/h3H,2H2,1H3,(H3,8,10,11,13) | |
Source | PubChem | |
URL | https://pubchem.ncbi.nlm.nih.gov | |
Description | Data deposited in or computed by PubChem | |
InChI Key |
WDOYBEPLTCFIRQ-UHFFFAOYSA-N | |
Source | PubChem | |
URL | https://pubchem.ncbi.nlm.nih.gov | |
Description | Data deposited in or computed by PubChem | |
Canonical SMILES |
CCN1C=NC2=C1N=C(NC2=O)N | |
Source | PubChem | |
URL | https://pubchem.ncbi.nlm.nih.gov | |
Description | Data deposited in or computed by PubChem | |
Molecular Formula |
C7H9N5O | |
Source | PubChem | |
URL | https://pubchem.ncbi.nlm.nih.gov | |
Description | Data deposited in or computed by PubChem | |
DSSTOX Substance ID |
DTXSID40236684 | |
Record name | 9-Ethylguanine | |
Source | EPA DSSTox | |
URL | https://comptox.epa.gov/dashboard/DTXSID40236684 | |
Description | DSSTox provides a high quality public chemistry resource for supporting improved predictive toxicology. | |
Molecular Weight |
179.18 g/mol | |
Source | PubChem | |
URL | https://pubchem.ncbi.nlm.nih.gov | |
Description | Data deposited in or computed by PubChem | |
CAS No. |
879-08-3 | |
Record name | 9-Ethylguanine | |
Source | ChemIDplus | |
URL | https://pubchem.ncbi.nlm.nih.gov/substance/?source=chemidplus&sourceid=0000879083 | |
Description | ChemIDplus is a free, web search system that provides access to the structure and nomenclature authority files used for the identification of chemical substances cited in National Library of Medicine (NLM) databases, including the TOXNET system. | |
Record name | 9-Ethylguanine | |
Source | DTP/NCI | |
URL | https://dtp.cancer.gov/dtpstandard/servlet/dwindex?searchtype=NSC&outputformat=html&searchlist=22755 | |
Description | The NCI Development Therapeutics Program (DTP) provides services and resources to the academic and private-sector research communities worldwide to facilitate the discovery and development of new cancer therapeutic agents. | |
Explanation | Unless otherwise indicated, all text within NCI products is free of copyright and may be reused without our permission. Credit the National Cancer Institute as the source. | |
Record name | 9-Ethylguanine | |
Source | EPA DSSTox | |
URL | https://comptox.epa.gov/dashboard/DTXSID40236684 | |
Description | DSSTox provides a high quality public chemistry resource for supporting improved predictive toxicology. | |
Record name | 9-ETHYLGUANINE | |
Source | FDA Global Substance Registration System (GSRS) | |
URL | https://gsrs.ncats.nih.gov/ginas/app/beta/substances/A31S01JPR6 | |
Description | The FDA Global Substance Registration System (GSRS) enables the efficient and accurate exchange of information on what substances are in regulated products. Instead of relying on names, which vary across regulatory domains, countries, and regions, the GSRS knowledge base makes it possible for substances to be defined by standardized, scientific descriptions. | |
Explanation | Unless otherwise noted, the contents of the FDA website (www.fda.gov), both text and graphics, are not copyrighted. They are in the public domain and may be republished, reprinted and otherwise used freely by anyone without the need to obtain permission from FDA. Credit to the U.S. Food and Drug Administration as the source is appreciated but not required. | |
Retrosynthesis Analysis
AI-Powered Synthesis Planning: Our tool employs the Template_relevance Pistachio, Template_relevance Bkms_metabolic, Template_relevance Pistachio_ringbreaker, Template_relevance Reaxys, Template_relevance Reaxys_biocatalysis model, leveraging a vast database of chemical reactions to predict feasible synthetic routes.
One-Step Synthesis Focus: Specifically designed for one-step synthesis, it provides concise and direct routes for your target compounds, streamlining the synthesis process.
Accurate Predictions: Utilizing the extensive PISTACHIO, BKMS_METABOLIC, PISTACHIO_RINGBREAKER, REAXYS, REAXYS_BIOCATALYSIS database, our tool offers high-accuracy predictions, reflecting the latest in chemical research and data.
Strategy Settings
Precursor scoring | Relevance Heuristic |
---|---|
Min. plausibility | 0.01 |
Model | Template_relevance |
Template Set | Pistachio/Bkms_metabolic/Pistachio_ringbreaker/Reaxys/Reaxys_biocatalysis |
Top-N result to add to graph | 6 |
Feasible Synthetic Routes
Disclaimer and Information on In-Vitro Research Products
Please be aware that all articles and product information presented on BenchChem are intended solely for informational purposes. The products available for purchase on BenchChem are specifically designed for in-vitro studies, which are conducted outside of living organisms. In-vitro studies, derived from the Latin term "in glass," involve experiments performed in controlled laboratory settings using cells or tissues. It is important to note that these products are not categorized as medicines or drugs, and they have not received approval from the FDA for the prevention, treatment, or cure of any medical condition, ailment, or disease. We must emphasize that any form of bodily introduction of these products into humans or animals is strictly prohibited by law. It is essential to adhere to these guidelines to ensure compliance with legal and ethical standards in research and experimentation.