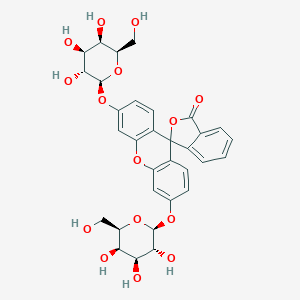
Fluorescein-digalactoside
Overview
Description
Fluorescein-digalactoside (FDG), also known as fluorescein di(β-D-galactopyranoside), is a fluorogenic substrate widely used in biochemical assays to detect β-galactosidase enzyme activity. Its molecular formula is C₃₂H₃₂O₁₅, with a molecular weight of 656.59 g/mol . Structurally, FDG consists of a fluorescein core conjugated to two β-D-galactopyranoside moieties via glycosidic bonds. The compound is non-fluorescent in its intact form due to quenching by the galactose residues. Upon enzymatic cleavage by β-galactosidase, the galactose groups are removed, releasing highly fluorescent fluorescein (excitation/emission: ~490/514 nm). This property makes FDG a critical tool in applications such as gene expression studies (e.g., lacZ reporter systems) and cell viability assays .
Preparation Methods
Synthetic Routes and Reaction Conditions
Fluorescein di-β-D-galactopyranoside can be synthesized through the glycosylation of fluorescein with galactose derivatives. The reaction typically involves the use of a glycosyl donor, such as galactose pentaacetate, and a catalyst like boron trifluoride etherate . The reaction is carried out in an anhydrous solvent, such as dichloromethane, under controlled temperature conditions to ensure high yield and purity.
Industrial Production Methods
Industrial production of fluorescein di-β-D-galactopyranoside follows similar synthetic routes but on a larger scale. The process involves rigorous purification steps, including crystallization and chromatography, to achieve the desired product quality. The compound is then stored under specific conditions to maintain its stability and activity .
Chemical Reactions Analysis
Types of Reactions
Fluorescein di-β-D-galactopyranoside primarily undergoes hydrolysis reactions catalyzed by β-galactosidase. This enzymatic reaction results in the sequential cleavage of the galactose moieties, first forming fluorescein monogalactoside and then fluorescein .
Common Reagents and Conditions
The hydrolysis reaction requires the presence of β-galactosidase and is typically carried out in a buffered aqueous solution at an optimal pH of around 7.0 . The reaction can be monitored by measuring the increase in fluorescence or absorbance.
Major Products
The major product of the hydrolysis reaction is fluorescein, a highly fluorescent compound that can be easily detected using fluorescence microscopy or flow cytometry .
Scientific Research Applications
Key Applications
-
Detection of β-Galactosidase Activity
- FDG serves as an ultra-sensitive substrate for detecting β-galactosidase, particularly in E. coli lacZ gene expression studies.
- It can be utilized in both in vivo and in vitro assays, allowing researchers to quantify enzymatic activity with high precision.
-
Flow Cytometry
- FDG is widely used in flow cytometry for quantifying β-galactosidase activity in gram-negative bacteria and mammalian cells. Its ability to penetrate viable cells enables real-time monitoring of enzyme activity .
- A comparative study indicated that while FDG effectively enters viable cells, its derivative C12-FDG showed limited penetration, highlighting the advantages of FDG in cellular assays .
- Cell Senescence Studies
- Lysosomal Storage Disease Research
- Gene Expression Analysis
Comparative Data Table
Application | Description | Advantages |
---|---|---|
Detection of β-Galactosidase | Sensitive substrate for lacZ gene expression | High sensitivity; suitable for live cell assays |
Flow Cytometry | Quantifies enzyme activity in viable cells | Real-time monitoring; versatile use |
Cell Senescence Studies | Measures senescence-associated β-galactosidase activity | Insights into aging processes |
Lysosomal Storage Disease Research | Investigates diseases linked to deficient lysosomal β-galactosidase activity | Potential for therapeutic insights |
Gene Expression Analysis | Detects β-galactosidase fusion proteins | Useful across various cell types |
Case Studies
-
Flow Cytometric Detection
A study demonstrated the efficiency of FDG in detecting β-galactosidase activity using flow cytometry across different cell types, including mammalian and bacterial cells. The results indicated that FDG provided a fluorescence signal directly proportional to enzymatic activity, facilitating accurate quantification . -
Senescence-Associated Activity Measurement
Researchers employed a fluorimetric method using FDG to quantify β-galactosidase activity associated with cellular senescence in human foreskin fibroblast (Hs68) cells. The findings underscored the utility of FDG in aging research, opening avenues for further exploration into cellular lifespan and health . -
Lysosomal Enzyme Activity Studies
The application of FDG derivatives was explored in the context of lysosomal storage diseases. By utilizing fluorescein-labeled substrates, researchers were able to visualize and quantify enzyme deficiencies linked to specific genetic disorders, providing critical insights into disease mechanisms and potential treatments .
Mechanism of Action
The mechanism of action of fluorescein di-β-D-galactopyranoside involves its hydrolysis by β-galactosidase. The enzyme cleaves the glycosidic bonds, releasing fluorescein monogalactoside and subsequently fluorescein . The fluorescence intensity of the resulting fluorescein is proportional to the enzymatic activity, allowing for quantitative analysis of β-galactosidase activity .
Comparison with Similar Compounds
Fluorescein-digalactoside belongs to a class of fluorogenic glycosides. Below, we compare its properties with structurally or functionally analogous compounds.
Structural and Functional Analogues
Fluorescein-Monogalactoside (FMG)
- Structure : Contains a single galactose residue linked to fluorescein.
- Enzymatic Cleavage : Requires one hydrolysis step by β-galactosidase to release fluorescein.
- Fluorescence Quenching : Less efficient quenching compared to FDG due to a single galactose group.
- Applications : Faster signal generation but higher background fluorescence than FDG.
Fluorescein-Di-glucoside (FDGlc)
- Structure: Two β-D-glucopyranoside groups instead of galactose.
- Molecular Weight : Similar to FDG (656.59 g/mol) but distinct sugar specificity.
Resorufin-β-D-galactopyranoside (RGP)
- Structure : Resorufin core linked to galactose.
- Fluorescence Properties : Higher excitation/emission wavelengths (~570/585 nm), reducing interference with autofluorescence in biological samples.
- Cleavage Efficiency : Single-step hydrolysis, yielding faster kinetics than FDG.
Quantitative Comparison
Key Findings from Comparative Studies
Enzyme Kinetics : FDG exhibits slower signal generation compared to FMG or RGP due to its two-step cleavage mechanism. However, its low background fluorescence enhances sensitivity in low-activity assays .
Specificity : FDG is highly specific to β-galactosidase, unlike FDGlc, which is tailored for β-glucosidase. This specificity reduces cross-reactivity in complex biological systems.
Photostability : Fluorescein derivatives like FDG are more photostable than resorufin-based substrates but require compatible excitation sources.
Biological Activity
Fluorescein-digalactoside (FDG) is a fluorogenic substrate widely used in biological research, particularly for detecting β-galactosidase activity. This compound is crucial in various applications, including cell biology, microbiology, and cancer research. This article explores the biological activity of FDG, its mechanisms, applications, and relevant case studies.
This compound is a nonfluorescent compound that becomes fluorescent upon hydrolysis by β-galactosidase. The hydrolysis process occurs in two steps:
- First Hydrolysis : FDG is hydrolyzed to fluorescein monogalactoside (FMG).
- Second Hydrolysis : FMG is further hydrolyzed to fluorescein, a highly fluorescent compound.
This reaction allows for the quantification of β-galactosidase activity through the measurement of fluorescence intensity, which correlates with enzymatic activity .
1. Detection of β-Galactosidase Activity
FDG is primarily used as a substrate for detecting β-galactosidase in various organisms, including bacteria and mammalian cells. The sensitivity of FDG makes it an ideal choice for flow cytometry and fluorescence microscopy. Studies have demonstrated its utility in:
- Identifying senescent cells : FDG can be used to detect senescence-associated β-galactosidase (SA-β-gal) activity, which is a marker for cellular aging .
- Analyzing recombinant proteins : Researchers utilize FDG to monitor the expression of β-galactosidase fusion proteins in genetically modified organisms .
2. Cellular Studies
FDG has been employed in various cellular studies to assess metabolic activity and cell viability. For instance:
- In cancer research , FDG has been utilized to study the metabolic differences between cancerous and normal cells by measuring β-galactosidase activity .
- In neurobiology , it has been used to investigate lysosomal storage diseases associated with deficient lysosomal β-galactosidase activity .
Case Study 1: Detection of Senescence in Human Cells
A study utilized FDG to quantify SA-β-gal activity in human foreskin fibroblast (Hs68) cells. The researchers developed a fluorimetric method that allowed for rapid quantification of senescence markers. The results indicated a significant increase in fluorescence intensity correlating with cellular senescence, demonstrating the effectiveness of FDG as a sensitive detection tool .
Case Study 2: Flow Cytometric Analysis
In another study, researchers employed FDG in flow cytometric analysis to sort viable cells expressing β-galactosidase. This method enabled the identification and isolation of specific cell populations based on their enzymatic activity, facilitating further studies on gene regulation at the single-cell level .
Comparative Analysis of Fluorogenic Substrates
The following table summarizes the characteristics of different fluorogenic substrates used for detecting β-galactosidase:
Substrate | Sensitivity | Application Area | Notes |
---|---|---|---|
This compound | High | Cell biology, microbiology | Widely used; sensitive to enzymatic activity |
C12-FDG | Moderate | Animal cells | Requires cell permeabilization for yeast |
DDAO galactoside | High | Neurochemistry | Detects longer wavelengths; less autofluorescence interference |
Q & A
Basic Research Questions
Q. What are the established protocols for synthesizing Fluorescein-digalactoside, and how can purity be validated?
this compound is typically synthesized via enzymatic or chemical glycosylation of fluorescein with galactose residues. Key steps include protecting group strategies to ensure regioselectivity and HPLC purification for isolating the product. Purity validation involves mass spectrometry (for molecular weight confirmation) and high-resolution NMR (to verify glycosidic bond formation). Quantitative analysis of residual solvents or unreacted substrates can be performed using GC-MS or UV-Vis spectrophotometry .
Q. Which spectroscopic techniques are most effective for detecting this compound in enzymatic assays?
Fluorescence spectroscopy (excitation/emission at 490/514 nm) is the primary method due to the compound’s inherent fluorescence. For kinetic studies, stopped-flow fluorescence assays provide real-time monitoring of galactosidase activity. UV-Vis spectrophotometry (at 492 nm) is a cost-effective alternative, though less sensitive. Confocal microscopy is used for spatial localization in cellular systems .
Q. What are the primary applications of this compound in studying glycosidase activity?
It serves as a fluorogenic substrate for β-galactosidases, enabling real-time tracking of enzyme kinetics in vitro and in vivo. Applications include screening enzyme inhibitors, profiling glycosidase activity in lysosomal storage disorders, and monitoring gene expression in lacZ-based reporter systems .
Q. How does the structure of this compound influence its specificity as a substrate for galactosidases?
The digalactoside moiety mimics natural substrates, ensuring recognition by β-galactosidases. Steric hindrance from the fluorescein group limits cross-reactivity with non-target enzymes. Structural analogs (e.g., mono- vs. di-galactosides) can be tested via competitive inhibition assays to validate specificity .
Advanced Research Questions
Q. How can researchers optimize experimental conditions to minimize photobleaching of this compound in live-cell imaging?
Use low-intensity light sources and antifade reagents (e.g., ascorbic acid). Two-photon microscopy reduces photobleaching by limiting excitation to the focal plane. Control experiments should quantify photobleaching rates under varying conditions (e.g., pH, temperature) to adjust imaging protocols .
Q. What statistical approaches are recommended for analyzing dose-response data involving this compound in kinetic studies?
Non-linear regression models (e.g., Michaelis-Menten or Hill equations) are standard for enzyme kinetics. Bootstrapping or Bayesian methods account for variability in replicates. For high-throughput data, machine learning algorithms (e.g., random forests) can identify outliers or non-linear trends .
Q. How should discrepancies in reported binding affinities of this compound across studies be addressed?
Conduct meta-analyses to identify methodological differences (e.g., buffer composition, temperature). Validate assays using standardized protocols (e.g., ISO guidelines) and reference compounds. Surface plasmon resonance (SPR) or isothermal titration calorimetry (ITC) provide orthogonal affinity measurements to resolve contradictions .
Q. What are the best practices for integrating this compound-based assays with other biochemical techniques to ensure data reproducibility?
Cross-validate results with orthogonal methods (e.g., ELISA for protein quantification or LC-MS for metabolite profiling). Use internal controls (e.g., spiked substrates) and inter-laboratory validation to address batch-to-batch variability. Document all experimental parameters (e.g., pH, ionic strength) in line with FAIR data principles .
Q. What methodological controls are essential when using this compound in high-throughput screening environments?
Include negative controls (e.g., substrate-only wells) to account for autofluorescence and positive controls (e.g., known inhibitors). Normalize fluorescence signals to plate-specific background readings. Use Z-factor analysis to assess assay robustness and minimize false positives .
Q. How can computational modeling be applied to predict the interaction dynamics of this compound with target enzymes?
Molecular docking (e.g., AutoDock Vina) predicts binding conformations, while molecular dynamics simulations (e.g., GROMACS) assess stability under physiological conditions. QSAR models correlate structural features with activity data to guide substrate optimization .
Q. Key Methodological Considerations
- Reproducibility : Follow guidelines from the Beilstein Journal of Organic Chemistry for detailed experimental reporting, including raw data deposition in repositories like Zenodo .
- Data Analysis : Use tools like R or Python for statistical modeling, ensuring code and datasets are openly accessible to facilitate peer review .
- Ethical Standards : Adhere to institutional protocols for handling fluorescent probes in biological systems, including cytotoxicity assessments .
Properties
IUPAC Name |
3',6'-bis[[(2S,3R,4S,5R,6R)-3,4,5-trihydroxy-6-(hydroxymethyl)oxan-2-yl]oxy]spiro[2-benzofuran-3,9'-xanthene]-1-one | |
---|---|---|
Source | PubChem | |
URL | https://pubchem.ncbi.nlm.nih.gov | |
Description | Data deposited in or computed by PubChem | |
InChI |
InChI=1S/C32H32O15/c33-11-21-23(35)25(37)27(39)30(45-21)42-13-5-7-17-19(9-13)44-20-10-14(43-31-28(40)26(38)24(36)22(12-34)46-31)6-8-18(20)32(17)16-4-2-1-3-15(16)29(41)47-32/h1-10,21-28,30-31,33-40H,11-12H2/t21-,22-,23+,24+,25+,26+,27-,28-,30-,31-/m1/s1 | |
Source | PubChem | |
URL | https://pubchem.ncbi.nlm.nih.gov | |
Description | Data deposited in or computed by PubChem | |
InChI Key |
ZTOBILYWTYHOJB-WBCGDKOGSA-N | |
Source | PubChem | |
URL | https://pubchem.ncbi.nlm.nih.gov | |
Description | Data deposited in or computed by PubChem | |
Canonical SMILES |
C1=CC=C2C(=C1)C(=O)OC23C4=C(C=C(C=C4)OC5C(C(C(C(O5)CO)O)O)O)OC6=C3C=CC(=C6)OC7C(C(C(C(O7)CO)O)O)O | |
Source | PubChem | |
URL | https://pubchem.ncbi.nlm.nih.gov | |
Description | Data deposited in or computed by PubChem | |
Isomeric SMILES |
C1=CC=C2C(=C1)C(=O)OC23C4=C(C=C(C=C4)O[C@H]5[C@@H]([C@H]([C@H]([C@H](O5)CO)O)O)O)OC6=C3C=CC(=C6)O[C@H]7[C@@H]([C@H]([C@H]([C@H](O7)CO)O)O)O | |
Source | PubChem | |
URL | https://pubchem.ncbi.nlm.nih.gov | |
Description | Data deposited in or computed by PubChem | |
Molecular Formula |
C32H32O15 | |
Source | PubChem | |
URL | https://pubchem.ncbi.nlm.nih.gov | |
Description | Data deposited in or computed by PubChem | |
DSSTOX Substance ID |
DTXSID20170431 | |
Record name | Fluorescein-digalactoside | |
Source | EPA DSSTox | |
URL | https://comptox.epa.gov/dashboard/DTXSID20170431 | |
Description | DSSTox provides a high quality public chemistry resource for supporting improved predictive toxicology. | |
Molecular Weight |
656.6 g/mol | |
Source | PubChem | |
URL | https://pubchem.ncbi.nlm.nih.gov | |
Description | Data deposited in or computed by PubChem | |
CAS No. |
17817-20-8 | |
Record name | Fluorescein-digalactoside | |
Source | ChemIDplus | |
URL | https://pubchem.ncbi.nlm.nih.gov/substance/?source=chemidplus&sourceid=0017817208 | |
Description | ChemIDplus is a free, web search system that provides access to the structure and nomenclature authority files used for the identification of chemical substances cited in National Library of Medicine (NLM) databases, including the TOXNET system. | |
Record name | Fluorescein-digalactoside | |
Source | EPA DSSTox | |
URL | https://comptox.epa.gov/dashboard/DTXSID20170431 | |
Description | DSSTox provides a high quality public chemistry resource for supporting improved predictive toxicology. | |
Retrosynthesis Analysis
AI-Powered Synthesis Planning: Our tool employs the Template_relevance Pistachio, Template_relevance Bkms_metabolic, Template_relevance Pistachio_ringbreaker, Template_relevance Reaxys, Template_relevance Reaxys_biocatalysis model, leveraging a vast database of chemical reactions to predict feasible synthetic routes.
One-Step Synthesis Focus: Specifically designed for one-step synthesis, it provides concise and direct routes for your target compounds, streamlining the synthesis process.
Accurate Predictions: Utilizing the extensive PISTACHIO, BKMS_METABOLIC, PISTACHIO_RINGBREAKER, REAXYS, REAXYS_BIOCATALYSIS database, our tool offers high-accuracy predictions, reflecting the latest in chemical research and data.
Strategy Settings
Precursor scoring | Relevance Heuristic |
---|---|
Min. plausibility | 0.01 |
Model | Template_relevance |
Template Set | Pistachio/Bkms_metabolic/Pistachio_ringbreaker/Reaxys/Reaxys_biocatalysis |
Top-N result to add to graph | 6 |
Feasible Synthetic Routes
Disclaimer and Information on In-Vitro Research Products
Please be aware that all articles and product information presented on BenchChem are intended solely for informational purposes. The products available for purchase on BenchChem are specifically designed for in-vitro studies, which are conducted outside of living organisms. In-vitro studies, derived from the Latin term "in glass," involve experiments performed in controlled laboratory settings using cells or tissues. It is important to note that these products are not categorized as medicines or drugs, and they have not received approval from the FDA for the prevention, treatment, or cure of any medical condition, ailment, or disease. We must emphasize that any form of bodily introduction of these products into humans or animals is strictly prohibited by law. It is essential to adhere to these guidelines to ensure compliance with legal and ethical standards in research and experimentation.