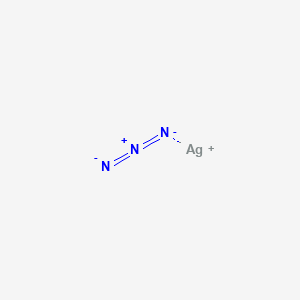
Silver azide
- Click on QUICK INQUIRY to receive a quote from our team of experts.
- With the quality product at a COMPETITIVE price, you can focus more on your research.
Overview
Description
Silver azide (AgN₃) is a highly sensitive primary explosive compound composed of silver and the azide ion (N₃⁻). It is synthesized by reacting silver nitrate (AgNO₃) with sodium azide (NaN₃) in aqueous solution, forming a white crystalline precipitate . Its crystal structure is orthorhombic, with a density of 4.42 g/cm³, and it detonates at a velocity of ~4,500 m/s upon initiation by heat, friction, or impact . However, its sensitivity and instability under certain conditions (e.g., exposure to light or ammonia) limit its widespread adoption .
Preparation Methods
Silver azide is typically synthesized through the reaction of silver nitrate with sodium azide. The reaction is carried out in an aqueous solution, where silver nitrate and sodium azide are mixed, resulting in the formation of silver monoazide as a precipitate. The reaction can be represented as follows:
AgNO3+NaN3→AgN3+NaNO3
In industrial production, the preparation of silver monoazide involves careful control of reaction conditions to ensure safety and consistency. The use of microreaction systems with integrated static micromixers has been shown to improve the crystal morphology and detonation behavior of silver monoazide, making the process safer and more efficient .
Chemical Reactions Analysis
Silver azide undergoes various chemical reactions, including:
-
Decomposition: : this compound decomposes explosively upon heating or impact, releasing nitrogen gas and forming metallic silver.
AgN3→Ag+3N2
-
Reaction with Acids: : this compound reacts with acids to form silver salts and hydrazoic acid.
AgN3+HCl→AgCl+HN3
-
Reaction with Bases: : this compound can react with bases to form silver hydroxide and sodium azide.
AgN3+NaOH→AgOH+NaN3
Scientific Research Applications
Explosives Technology
Primary Explosive
Silver azide is primarily known for its use as a primary explosive . It decomposes explosively upon heating or impact, releasing nitrogen gas and solid silver:
The decomposition temperature for pure this compound is approximately 340 °C, but this can be lowered to 270 °C in the presence of impurities . Due to its high sensitivity to shock and heat, it is utilized in detonators and as a primer in ammunition .
Catalysis in Organic Synthesis
Silver-Catalyzed Reactions
Recent studies have highlighted the potential of this compound as a catalyst in organic synthesis, particularly in the azide-alkyne cycloaddition reaction . This reaction is crucial for forming triazole compounds, which have significant applications in medicinal chemistry .
Case Study: Azide-Alkyne Cycloaddition
A study demonstrated the effectiveness of various silver salts, including this compound, as catalysts for this cycloaddition. The results showed that:
Silver Salt | Yield (%) | Reaction Conditions |
---|---|---|
AgOAc | 44 | TEA (5 equiv.) |
Ag₂O | 32 | TEA (5 equiv.) |
AgNO₃ | 13 | TEA (5 equiv.) |
Ag₂CO₃ | 61 | TEA (5 equiv.) |
AgCl | 87 | TEA (5 equiv.) |
AgI | 45 | TEA (5 equiv.) |
The highest yield was achieved with silver chloride, indicating that while this compound can be effective, other silver salts may provide better results under certain conditions .
Biomedical Applications
Explosive Nephrolithopaxy
this compound has been investigated for use in explosive nephrolithopaxy , a minimally invasive technique for breaking down kidney stones using explosive charges. Research has shown that charges of this compound can effectively fragment renal calculi when detonated . This application highlights the compound's potential beyond traditional explosive uses.
Safety Considerations
Given its explosive nature, handling this compound requires stringent safety measures. Decomposition can be triggered by exposure to light or physical shock, necessitating careful storage and handling protocols to prevent accidental detonation .
Mechanism of Action
The explosive nature of silver monoazide is primarily due to the rapid decomposition of the azide group, which releases a large volume of nitrogen gas. This rapid gas release generates a significant amount of pressure and heat, leading to an explosive reaction. The molecular targets and pathways involved in this process are related to the breaking of the nitrogen-nitrogen bonds within the azide group, resulting in the formation of nitrogen gas and metallic silver.
Comparison with Similar Compounds
Silver Azide vs. Lead Azide
Structural and Functional Comparison
- Chemical Stability : Lead azide is thermally stable up to 350°C, whereas this compound decomposes at ~250°C, making it less suitable for high-temperature applications .
- Sensitivity : this compound is more sensitive to friction and impact than lead azide, increasing handling risks .
- Environmental Impact : Lead azide poses significant environmental and health hazards due to lead content, driving research into this compound as a "greener" alternative .
Table 1: Comparative Properties of this compound and Lead Azide
Property | This compound (AgN₃) | Lead Azide (Pb(N₃)₂) |
---|---|---|
Density (g/cm³) | 4.42 | 4.71 |
Detonation Velocity (m/s) | ~4,500 | ~5,100 |
Decomposition Temp (°C) | 250 | 350 |
Sensitivity to Friction | High | Moderate |
Toxicity | Low | High (Pb contamination) |
Primary Use | Niche detonators | Standard detonators |
This compound vs. Sodium Azide
Structural Similarity, Functional Divergence
- Chemical Composition: Both contain the azide ion, but sodium azide (NaN₃) is a non-explosive ionic compound used in airbag inflators and biocides .
- Reactivity : Sodium azide decomposes to release nitrogen gas (N₂) upon heating, whereas this compound detonates explosively due to the rapid release of energy from Ag-N bond cleavage .
- Toxicity : Sodium azide is highly toxic (LD₅₀: 27 mg/kg in rats), whereas this compound’s primary hazard is its explosiveness .
This compound vs. Other Silver Salts
Silver halides (e.g., AgBr, AgI) and silver acetate (AgC₂H₃O₂) share structural motifs with this compound but lack explosive properties:
- Silver Iodide (AgI): Used in cloud seeding and photography; stable under ambient conditions and non-detonative .
- Silver Acetate : A mild oxidizing agent with applications in organic synthesis; decomposes at 220°C without explosion .
Table 2: Functional Comparison of Silver Compounds
Compound | Primary Use | Reactivity | Hazard Profile |
---|---|---|---|
This compound | Explosives | High (explosive) | Sensitivity to shock |
Silver Iodide | Cloud seeding, photography | Low | Low toxicity |
Silver Acetate | Organic synthesis | Moderate (oxidizer) | Irritant |
Research Findings and Critical Analysis
- Synthesis Advancements : this compound production has been optimized to reduce sensitivity by controlling crystal size and morphology during precipitation .
- Environmental Trade-offs : While this compound avoids lead toxicity, silver’s environmental persistence raises concerns about long-term ecosystem impacts .
- Performance Limitations : Despite its high detonation velocity, this compound’s instability under humid conditions limits its adoption in military applications compared to lead azide .
Q & A
Q. Basic: What are the critical parameters to consider when synthesizing silver azide to ensure purity and reproducibility?
Answer:
Synthesis of this compound (AgN₃) requires precise control of stoichiometry, reaction temperature (typically 0–5°C), and pH (maintained at ~6–7 using nitric acid). Key steps include:
- Precipitation method : Gradual addition of sodium azide (NaN₃) to silver nitrate (AgNO₃) under controlled agitation .
- Characterization : Use X-ray diffraction (XRD) to confirm crystal structure and energy-dispersive X-ray spectroscopy (EDS) for elemental composition. Purity is validated via differential scanning calorimetry (DSC) to detect impurities that alter decomposition thresholds .
- Safety : Conduct reactions in fume hoods with blast shields due to AgN₃’s extreme sensitivity .
Q. Advanced: How can computational models resolve discrepancies in reported activation energies for this compound decomposition?
Answer:
Conflicting activation energy values (e.g., 120–150 kJ/mol in thermal studies vs. 80–100 kJ/mol in shock-induced decomposition) arise from differing experimental conditions. To address this:
- Multi-scale modeling : Combine density functional theory (DFT) for electronic structure analysis with molecular dynamics (MD) simulations to model thermal vs. mechanical stimuli .
- Experimental validation : Cross-reference computational predictions with time-resolved mass spectrometry under controlled heating rates (e.g., 5–20°C/min) and high-pressure setups .
- Error analysis : Quantify uncertainties in DSC measurements (e.g., ±2% for enthalpy) and shockwave calibration .
Q. Basic: What spectroscopic techniques are most effective for characterizing this compound’s structural stability?
Answer:
- Raman spectroscopy : Identifies N₃⁻ vibrational modes (asymmetric stretch at ~2100 cm⁻¹) to detect lattice distortions .
- X-ray photoelectron spectroscopy (XPS) : Monitors silver oxidation states (Ag⁰ vs. Ag⁺) to assess surface degradation .
- FTIR : Tracks azide group stability under humidity (peaks at 640 cm⁻¹ for N–N bonds) .
Q. Advanced: How do crystal defects influence this compound’s sensitivity, and what experimental designs can quantify this relationship?
Answer:
Crystal defects (e.g., vacancies, dislocations) lower activation energy by creating localized stress points. Methodologies include:
- Defect engineering : Introduce controlled defects via rapid crystallization or doping (e.g., 0.1% Cu²⁺) and analyze via high-resolution transmission electron microscopy (HRTEM) .
- Sensitivity testing : Use a fall-hammer apparatus (e.g., 0.5–2 J impact energy) to correlate defect density with initiation thresholds .
- Data interpretation : Apply Weibull statistics to model probabilistic relationships between defect distribution and sensitivity .
Q. Basic: What are the best practices for handling and storing this compound to minimize accidental detonation?
Answer:
- Storage : Keep in amber glass vials under argon at −20°C to inhibit moisture absorption and photolytic decomposition .
- Handling : Use non-sparking tools (e.g., Teflon-coated) and avoid friction/static electricity. Conduct sensitivity tests (e.g., BAM friction test) for batch-specific risk assessment .
Q. Advanced: How can isotopic labeling (e.g., ¹⁵N) clarify decomposition pathways in this compound?
Answer:
- Synthesis of ¹⁵N-labeled AgN₃ : React AgNO₃ with NaN₃ enriched to 99% ¹⁵N .
- Mechanistic studies : Use isotope-ratio mass spectrometry (IRMS) to track nitrogen release (N₂ vs. N₃ radicals) during thermal decomposition. Compare with DFT-predicted pathways .
Q. Basic: What factors contribute to contradictory reports on this compound’s solubility in polar solvents?
Answer:
Discrepancies arise from solvent purity and measurement techniques. To standardize:
- Solubility testing : Use UV-Vis spectroscopy (λ = 300 nm for Ag⁺ detection) in deionized water at 25°C. Report results as g/100 mL ± SEM .
- Control variables : Pre-filter solvents (0.22 µm) to remove particulates that catalyze decomposition .
Q. Advanced: What methodologies enable real-time monitoring of this compound’s decomposition kinetics under varying pressures?
Answer:
- High-pressure DSC : Operate at 1–10 MPa with sapphire windows to observe phase transitions .
- Synchrotron XRD : Capture lattice dynamics during shock compression (time resolution < 1 µs) .
- Data normalization : Correct for pressure-induced thermal conductivity changes using reference materials (e.g., KCl) .
Q. Basic: How do researchers mitigate interference from byproducts when analyzing this compound’s explosive properties?
Answer:
- Chromatographic separation : Use HPLC with a C18 column to isolate AgN₃ from decomposition byproducts (e.g., AgNH₂) .
- Calibration curves : Validate purity via comparison with NIST-certified AgN₃ standards .
Q. Advanced: What gaps exist in understanding this compound’s photolytic behavior, and how can they be addressed experimentally?
Answer:
- Knowledge gaps : Role of UV wavelength (e.g., 254 nm vs. 365 nm) on decomposition rates and quantum yield variability.
- Experimental design : Use monochromatic light sources coupled with time-resolved FTIR to track intermediate species (e.g., AgN₂⁺) .
- Collaborative studies : Cross-reference photolytic data with computational models of exciton formation .
Properties
CAS No. |
13863-88-2 |
---|---|
Molecular Formula |
AgN3 |
Molecular Weight |
149.889 g/mol |
IUPAC Name |
silver;azide |
InChI |
InChI=1S/Ag.N3/c;1-3-2/q+1;-1 |
InChI Key |
QBFXQJXHEPIJKW-UHFFFAOYSA-N |
SMILES |
[N-]=[N+]=[N-].[Ag+] |
Canonical SMILES |
[N-]=[N+]=[N-].[Ag+] |
Key on ui other cas no. |
13863-88-2 |
Pictograms |
Explosive |
Synonyms |
silver azide |
Origin of Product |
United States |
Disclaimer and Information on In-Vitro Research Products
Please be aware that all articles and product information presented on BenchChem are intended solely for informational purposes. The products available for purchase on BenchChem are specifically designed for in-vitro studies, which are conducted outside of living organisms. In-vitro studies, derived from the Latin term "in glass," involve experiments performed in controlled laboratory settings using cells or tissues. It is important to note that these products are not categorized as medicines or drugs, and they have not received approval from the FDA for the prevention, treatment, or cure of any medical condition, ailment, or disease. We must emphasize that any form of bodily introduction of these products into humans or animals is strictly prohibited by law. It is essential to adhere to these guidelines to ensure compliance with legal and ethical standards in research and experimentation.