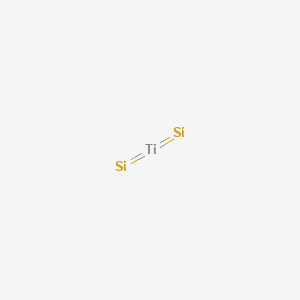
Titanium disilicide
Overview
Description
Titanium disilicide (TiSi₂) is a refractory metal silicide with the chemical formula TiSi₂. It exhibits a molar mass of 104.038 g/mol, a density of 4.02 g/cm³, and a melting point reported between 1470 °C and 1500 °C . Its orthorhombic crystalline structure exists in two phases: the metastable C49 phase (formed below 700 °C) and the stable C54 phase (formed above 700 °C), the latter of which has superior electrical properties . TiSi₂ is notable for its exceptionally low room-temperature electrical resistivity (13–16 μΩ·cm), the lowest among transition metal silicides, making it a preferred material for interconnects in integrated circuits .
Thermodynamically, TiSi₂ has a Debye temperature of ~510 K and follows a Dulong-Petit specific heat trend above 200 K, with applications in high-temperature environments . It is synthesized via methods such as self-propagating high-temperature combustion , aluminothermic reduction , and chemical vapor deposition . Industrially, TiSi₂ is utilized in biosensors (enhancing plasmon resonance sensitivity ), ceramic composites (improving fracture toughness and electrical conductivity ), and photocatalysis (water-splitting ).
Preparation Methods
Direct Solid-State Reaction
The most straightforward method for synthesizing TiSi₂ involves the direct reaction of titanium (Ti) or titanium hydride (TiH₂) with silicon (Si) at elevated temperatures. Stoichiometric mixtures of Ti and Si powders are heated in vacuum or inert atmospheres (e.g., argon) above 1,000°C . The reaction proceeds as:
This method yields the C49 phase of TiSi₂, a metastable orthorhombic structure with higher resistivity (60–100 μΩ·cm) . Post-annealing at 800–900°C facilitates transformation to the thermodynamically stable C54 phase, which exhibits superior conductivity (12–20 μΩ·cm) . Challenges include incomplete homogenization and residual impurities, necessitating stringent stoichiometric control .
Aluminothermic Reduction
Aluminothermic reduction leverages the exothermic reaction between aluminum (Al), sulfur (S), and metal oxides to produce TiSi₂. A typical mixture includes Al powder, S, silicon dioxide (SiO₂), and titanium dioxide (TiO₂) or potassium hexafluorotitanate (K₂TiF₆) . Ignition initiates the reaction:
This method achieves high-purity TiSi₂ but requires careful handling of reactive intermediates. The use of K₂TiF₆ enhances reaction efficiency by lowering the activation energy .
Electrolytic Deposition
Electrolysis of molten salts containing titanium and silicon precursors offers a scalable route for TiSi₂ synthesis. A common electrolyte comprises potassium hexafluorotitanate (K₂TiF₆) and TiO₂, which are electrolyzed at 900–1,000°C . Titanium migrates to the cathode, reacting with silicon to form TiSi₂:
This method is advantageous for producing thin films but demands precise control over current density and temperature .
Gas-Phase Reactions
Silicon Tetrachloride Route
Titanium tetrachloride (TiCl₄) reacts with silicon tetrachloride (SiCl₄) or silicon (Si) in hydrogen atmospheres:
This vapor-phase deposition technique is suitable for coating substrates but generates corrosive byproducts .
Silane-Based Synthesis
Silane (SiH₄) or dichlorosilane (SiH₂Cl₂) reduces TiCl₄ at 500–700°C:
This method produces high-purity TiSi₂ films, ideal for semiconductor applications .
Chemical Vapor Deposition (CVD)
CVD is widely used to deposit TiSi₂ thin films on silicon wafers. Titanium chloride (TiCl₄) and silane (SiH₄) are introduced into a reactor at 600–800°C, where they react on heated substrates :
CVD enables precise thickness control and uniform coatings but requires high vacuum and costly equipment .
Mechanical Alloying and Self-Propagating High-Temperature Synthesis (SHS)
Mechanical alloying involves high-energy ball milling of Ti and Si powders, inducing a mechanically driven self-propagating reaction (MSR). For example, milling 5Ti + 3Si forms Ti₅Si₃, while Ti + 2Si yields TiSi₂ after an induction period . Subsequent annealing converts metastable phases to C54-TiSi₂.
SHS utilizes the exothermicity of Ti-Si reactions, ignited by thermal or shock stimuli. While rapid, this method often produces multiphase products requiring post-processing .
In-Situ Synthesis in Composite Materials
TiSi₂ reinforcements are synthesized in situ within matrices like Ti₃AlC₂. A mixture of Ti, Al, Si, and graphite powders (molar ratio 3:1.1:0.2–0.8:2) is sintered at 1,400°C under argon . The reaction proceeds as:
This approach enhances mechanical properties but complicates phase purity .
Comparative Analysis of Synthesis Methods
Method | Conditions | Phase | Resistivity (μΩ·cm) | Advantages | Limitations |
---|---|---|---|---|---|
Direct Reaction | 1,000°C, Argon | C49/C54 | 60–100 / 12–20 | Simple, scalable | Impurities, phase control |
Aluminothermic Reduction | Exothermic ignition | C54 | 15–25 | High purity | Hazardous intermediates |
CVD | 600–800°C, Vacuum | C54 | 12–20 | Thin film uniformity | High cost, equipment-intensive |
Mechanical Alloying | Ball milling + Annealing | C54 | 15–25 | Nanostructured materials | Time-consuming |
Challenges in Phase Control and Industrial Applications
Achieving the C54 phase remains a critical challenge due to its higher activation energy. Strategies include:
-
Post-Deposition Annealing : Heating C49-TiSi₂ at 800–900°C induces phase transition .
-
Doping : Incorporating elements like Mo or W stabilizes the C54 phase .
In semiconductor manufacturing, C54-TiSi₂ is preferred for gate electrodes and interconnects due to its low resistivity .
Chemical Reactions Analysis
Direct Reaction of Titanium and Silicon
The simplest method involves heating titanium and silicon in stoichiometric ratios:
This exothermic reaction occurs at high temperatures (typically >700°C) and is used for bulk synthesis .
Aluminothermic Reduction
A redox reaction using aluminum powder as a reducing agent:
This method is cost-effective for large-scale production but requires precise control of ignition conditions .
Reactions with Titanium Tetrachloride (TiCl₄)
TiCl₄ reacts with silane (SiH₄) or dichlorosilane (SiH₂Cl₂) in gas-phase processes:
These reactions are favored in chemical vapor deposition (CVD) for thin-film applications .
Phase Transitions and Stability
TiSi₂ exhibits two polymorphs: C49 (high-resistivity) and C54 (low-resistivity). Phase transitions are critical for semiconductor performance.
Property | C49 Phase | C54 Phase |
---|---|---|
Resistivity (μΩ·cm) | 60–70 | 15–20 |
Formation Temperature | 600–750°C | 800–900°C |
Stability | Metastable | Thermodynamically stable |
-
C49-to-C54 Transition :
Driven by annealing at 800–900°C . Heavy doping (e.g., As or P) raises the transition temperature (up to 850°C for As-doped substrates) .
The activation energy for this transition is ~3.5 eV, influenced by grain size and dopant distribution . -
Decomposition :
At temperatures >900°C, TiSi₂ decomposes into Ti₅Si₃ and Si:
This limits its use in high-temperature applications .
Oxidation and Hydrolysis
-
Reacts with steam at elevated temperatures:
This reaction is suppressed in inert atmospheres . -
Violent oxidation occurs with strong oxidizers (e.g., KNO₃), producing TiO₂ and SiO₂ .
Halogenation
TiSi₂ reacts with halogens at high temperatures:
Similar reactions occur with Cl₂, Br₂, and I₂, forming respective titanium halides .
Doping Effects
-
Arsenic-doped Si substrates : Form TiAs precipitates at C49 grain boundaries, increasing transition temperatures .
-
Phosphorus-doped substrates : No phosphide formation; transition temperatures remain stable (~800°C) .
Salicide Process
In semiconductor fabrication, Ti reacts with Si to form TiSi₂ via:
Key steps include:
-
C49 Formation : Initial reaction at 600–700°C.
-
C54 Transition : Annealing at 800–900°C for low-resistivity contacts .
Composite Formation
In Mo-Si-Ti systems, TiSi₂ forms solid solutions:
Mechanical activation (e.g., ball milling) enhances reaction kinetics and refines microstructures .
Reaction Kinetics and Thermodynamics
-
Activation Energy : ~200 kJ/mol for Ti-Si interdiffusion in thin films .
-
Adiabatic Temperature : 2550 K for Ti₅Si₃ formation, enabling self-propagating high-temperature synthesis (SHS) .
Reaction | ΔH (kJ/mol) | T (°C) |
---|---|---|
Ti + 2Si → TiSi₂ | -579 | >700 |
3TiO₂ + 6SiO₂ + 10Al → ... | -4200 | 1000 |
TiCl₄ + 2SiH₄ → TiSi₂ + ... | -320 | 400–600 |
Challenges and Mitigation
Scientific Research Applications
Microelectronics
VLSI Applications
Titanium disilicide is extensively used in Very Large Scale Integration (VLSI) technology as a contact material. It serves as an effective gate-level interconnect in silicon-based devices, enhancing electrical performance due to its low resistivity. Research indicates that TiSi₂ can be formed through various methods such as sputtering and chemical vapor deposition, which are critical for creating reliable interconnections in integrated circuits .
Thin Films for Semiconductor Devices
The formation of this compound thin films is crucial for semiconductor applications. These films exhibit excellent thermal and chemical stability, making them suitable for high-temperature environments often encountered in semiconductor processing. The deposition techniques employed include radio-frequency plasma-enhanced chemical vapor deposition, which facilitates the growth of high-quality TiSi₂ films on silicon substrates .
Energy Storage
Lithium-Ion Batteries
Recent studies have shown that this compound can be utilized as an anode material in lithium-ion batteries. Its incorporation into silicon nanonets significantly improves the capacity and cycling stability of the batteries. The TiSi₂-based anodes demonstrate a capacity retention of only 0.1% fade per cycle over numerous charge-discharge cycles, indicating their potential for next-generation energy storage solutions .
Catalysis
Photocatalytic Applications
this compound has emerged as a promising photocatalyst for various chemical reactions, especially in environmental applications such as water splitting and pollutant degradation. Research indicates that TiSi₂ can be combined with other materials to enhance photocatalytic efficiency, making it a valuable component in sustainable energy technologies .
Material Science
Composite Materials
In material science, this compound is being explored for its potential to form composite materials that leverage its mechanical strength and thermal stability. These composites are being investigated for applications in aerospace and automotive industries where lightweight and durable materials are essential.
-
VLSI Technology Development
A study conducted at the University of Surrey demonstrated the feasibility of using this compound in VLSI processes, highlighting its effectiveness in enhancing device performance through improved interconnect reliability . -
Battery Performance Enhancement
Research at Boston College showcased the development of silicon-coated this compound nanonets as an advanced anode material for lithium-ion batteries, achieving significant improvements in energy capacity and cycle life compared to traditional materials . -
Photocatalytic Efficiency
Investigations into this compound's role in photocatalysis have revealed its ability to absorb light effectively, thus facilitating chemical reactions under solar irradiation, which is vital for sustainable environmental solutions .
Mechanism of Action
The mechanism of action of titanium disilicide in semiconductor applications involves its ability to form low-resistivity contacts and reduce the sheet resistance of silicon and polysilicon lines . In photocatalysis, this compound acts as a catalyst, facilitating the splitting of water molecules under solar radiation .
Comparison with Similar Compounds
TiSi₂ is compared to other transition metal disilicides (e.g., TaSi₂, WSi₂, MoSi₂, ZrSi₂, HfSi₂) in terms of physical, thermal, and functional properties.
Table 1: Comparative Properties of Transition Metal Disilicides
Key Findings:
Thermal Stability :
- TaSi₂ and WSi₂ exhibit superior melting points (>2100 °C) compared to TiSi₂ (~1500 °C), making them suitable for ultra-high-temperature environments .
- MoSi₂ is uniquely stable up to 1700 °C in oxidizing conditions due to a protective SiO₂ layer, unlike TiSi₂, which degrades above 800 °C .
Electrical Properties :
- TiSi₂ outperforms all listed disilicides in electrical conductivity (13–16 μΩ·cm), critical for microelectronic interconnects .
- MoSi₂ has variable resistivity (21–100 μΩ·cm) depending on stoichiometry, limiting its use in precision electronics .
Mechanical Properties :
- TaSi₂ and WSi₂ exhibit higher hardness (15.6 GPa and 13.1 GPa, respectively) than TiSi₂ (8.6 GPa), favoring wear-resistant coatings .
- TiSi₂ enhances fracture toughness in composites (e.g., HA-TiSi₂: 1.2 MPa·m¹/² vs. HA: 0.6 MPa·m¹/²) via crack deflection mechanisms .
Functional Applications: Biosensors: TiSi₂-graphene heterostructures achieve 183.4°/RIU sensitivity, outperforming conventional Ag-based sensors . Energy: TiSi₂ nanostructures enable efficient charge separation in photocatalysis, unlike TaSi₂ or WSi₂ . Barrier Layers: TiSi₂/TiB₂ bilayers prevent boron diffusion in semiconductors, a niche application unmatched by ZrSi₂ or HfSi₂ .
Critical Notes on TiSi₂
- Synthesis Challenges : Phase control during synthesis (C49 vs. C54) is critical for optimizing resistivity .
- Safety : TiSi₂ is a flammable solid (H228) requiring stringent handling protocols .
- Trade-offs : While TiSi₂ excels in conductivity, its lower thermal stability limits high-temperature applications compared to MoSi₂ or WSi₂ .
Biological Activity
Titanium disilicide (TiSi₂) is an intermetallic compound that has garnered attention for its unique properties and potential applications in various fields, including electronics and materials science. Recent studies have also begun to explore its biological activity, particularly its interactions with biomolecules and cellular processes. This article provides a comprehensive overview of the biological activity of this compound, supported by data tables and relevant research findings.
This compound is characterized by its high melting point (approximately 2130 °C) and excellent oxidation resistance. It typically forms a complex hexagonal crystal structure, which contributes to its mechanical strength and stability at elevated temperatures. The synthesis of this compound can be achieved through various methods, including chemical vapor deposition, mechanical alloying, and self-propagating high-temperature synthesis (SHS) .
Research indicates that this compound can influence cellular processes by modulating cell signaling pathways and gene expression related to oxidative stress and apoptosis. The compound interacts with enzymes and proteins through metallic-covalent and ionic bonds, which can either inhibit or enhance their activities . These interactions suggest that this compound may play a role in biochemical processes involving oxidative stress responses.
In Vitro Studies
In vitro studies have demonstrated that this compound can affect various cell types. For instance, it has been shown to influence the proliferation and viability of specific cell lines, indicating potential cytotoxic effects at certain concentrations. These effects are believed to be mediated through oxidative stress mechanisms, where the compound may induce reactive oxygen species (ROS) production .
Case Studies
- Cellular Response to this compound :
- Biocompatibility Assessment :
Table 1: Summary of Biological Effects of this compound
Toxicological Considerations
Despite the promising applications of this compound in biomaterials, comprehensive toxicological assessments are still needed to fully understand its biological implications. Current data suggest that while there are beneficial interactions with certain cell types, there may also be cytotoxic effects at elevated concentrations . Further research is required to elucidate the long-term effects and mechanisms underlying these interactions.
Q & A
Basic Research Questions
Q. What are the primary synthesis methods for titanium disilicide (TiSi₂) in academic research, and how do their experimental parameters influence material properties?
TiSi₂ is synthesized via methods like the "chemical oven" self-propagating combustion (using Si and Ti in a 3:5 atomic ratio) and solid-state diffusion (e.g., depositing Ti onto Si followed by thermal reaction) . Key parameters include ignition temperature, reactant stoichiometry, and annealing duration. For instance, combustion synthesis yields high-purity TiSi₂ with controlled electrical resistivity (~13–16 μΩcm), while diffusion methods optimize thin-film formation for integrated circuits. Methodological adjustments, such as pre-compaction of elemental powders or controlled atmosphere annealing, directly impact phase purity and crystallinity.
Q. How can researchers characterize the crystal structure and phase purity of this compound (TiSi₂) post-synthesis?
X-ray diffraction (XRD) is standard for identifying the orthorhombic C54 phase of TiSi₂, while transmission electron microscopy (TEM) resolves nanoscale crystallinity and defects . Energy-dispersive X-ray spectroscopy (EDS) confirms stoichiometry, and Raman spectroscopy detects secondary phases like TiO₂ or unreacted Si. For thin films, grazing-incidence XRD and cross-sectional SEM are critical to assess interfacial uniformity .
Q. What are the key physical and electronic properties of this compound (TiSi₂) that make it suitable for high-temperature applications?
TiSi₂ exhibits a high melting point (1,470°C), density (~4.02 g/cm³), and thermal stability up to 1,800 K, making it viable for refractory applications . Its low electrical resistivity (~13–16 μΩcm) and corrosion resistance under oxidative environments are advantageous for interconnects in microelectronics . High-temperature stability is attributed to the formation of protective SiO₂ layers during oxidation, though kinetics vary with porosity and impurity content .
Advanced Research Questions
Q. What methodological approaches are used to analyze interfacial reactions between TiSi₂ and reinforcement materials in composite systems?
Thermochemical modeling (e.g., CALPHAD or DFT simulations) predicts compatibility between TiSi₂ and reinforcements like SiC, TiB₂, or ZrO₂ . Experimental validation involves high-temperature annealing followed by TEM-EDS to map elemental diffusion and interfacial reaction products. For example, SiC remains stable in TiSi₂ matrices up to 1,800 K, while carbon degrades due to SiO₂ formation .
Q. How can researchers resolve contradictions in reported electrical resistivity values of TiSi₂ across studies?
Discrepancies often arise from synthesis conditions (e.g., combustion vs. diffusion methods) or impurity phases. Methodological solutions include:
- Standardizing resistivity measurements via four-point probe techniques under inert atmospheres.
- Using TEM to identify secondary phases (e.g., TiO₂) that alter conductivity .
- Correlating stoichiometry (via EDS) with resistivity trends to isolate compositional effects.
Q. What experimental design considerations are critical when integrating TiSi₂ into surface plasmon resonance (SPR) biosensors?
Optimizing TiSi₂-graphene heterostructures requires precise layer thickness control (e.g., 45 nm Ag, 2 nm TiSi₂, 1.7 nm graphene) to enhance sensitivity . Attenuated total reflection (ATR) methods validate refractive index changes, while finite-difference time-domain (FDTD) simulations model plasmonic interactions. Key challenges include minimizing TiSi₂ oxidation during fabrication and ensuring graphene adhesion via van der Waals forces.
Q. What are the challenges in maintaining stoichiometric control during TiSi₂ synthesis via self-propagating combustion?
Rapid exothermic reactions can lead to localized Si evaporation or incomplete Ti-Si intermixing. Mitigation strategies include:
- Pre-milling reactants to reduce particle size and enhance homogeneity.
- Using inert gas atmospheres to suppress oxidation.
- Post-annealing at 800–1,000°C to homogenize phase distribution .
Q. How does silicon diffusion kinetics through TiSi₂ layers affect thin-film formation in integrated circuits?
During Ti-Si interdiffusion, TiSi₂ layer growth follows parabolic kinetics (thickness ∝ √time), with Si diffusion as the rate-limiting step . In situ XRD or spectroscopic ellipsometry monitors phase evolution, while Arrhenius analysis quantifies activation energies (~2–3 eV). Deviations from ideal kinetics may indicate interfacial contamination or stress-induced defects.
Q. What advanced techniques assess the thermal stability and oxidation resistance of TiSi₂ under extreme conditions?
Thermogravimetric analysis (TGA) quantifies mass loss during oxidation, while X-ray photoelectron spectroscopy (XPS) identifies surface oxide compositions (e.g., SiO₂ vs. TiO₂) . High-resolution TEM reveals protective SiO₂ layer integrity after cyclic thermal testing. Computational models (e.g., density functional theory) predict oxygen adsorption energies on TiSi₂ surfaces to guide alloying strategies (e.g., Al additions) .
Q. How can computational modeling complement experimental studies in predicting TiSi₂ compatibility with ceramic matrix composites?
Phase-field modeling simulates TiSi₂-SiC interfacial reactions under thermal gradients, while molecular dynamics (MD) evaluates mechanical properties (e.g., fracture toughness) . Coupling these with experimental data (e.g., nanoindentation) validates predictive accuracy. For example, MD predicts ZrO₂ reinforcements improve crack resistance in TiSi₂ composites, which is confirmed via micro-pillar compression tests.
Properties
InChI |
InChI=1S/2Si.Ti | |
---|---|---|
Source | PubChem | |
URL | https://pubchem.ncbi.nlm.nih.gov | |
Description | Data deposited in or computed by PubChem | |
InChI Key |
DFJQEGUNXWZVAH-UHFFFAOYSA-N | |
Source | PubChem | |
URL | https://pubchem.ncbi.nlm.nih.gov | |
Description | Data deposited in or computed by PubChem | |
Canonical SMILES |
[Si]=[Ti]=[Si] | |
Source | PubChem | |
URL | https://pubchem.ncbi.nlm.nih.gov | |
Description | Data deposited in or computed by PubChem | |
Molecular Formula |
TiSi2, Si2Ti | |
Record name | Titanium disilicide | |
Source | Wikipedia | |
URL | https://en.wikipedia.org/wiki/Titanium_disilicide | |
Description | Chemical information link to Wikipedia. | |
Source | PubChem | |
URL | https://pubchem.ncbi.nlm.nih.gov | |
Description | Data deposited in or computed by PubChem | |
DSSTOX Substance ID |
DTXSID70894998 | |
Record name | Titanium disilicide | |
Source | EPA DSSTox | |
URL | https://comptox.epa.gov/dashboard/DTXSID70894998 | |
Description | DSSTox provides a high quality public chemistry resource for supporting improved predictive toxicology. | |
Molecular Weight |
104.04 g/mol | |
Source | PubChem | |
URL | https://pubchem.ncbi.nlm.nih.gov | |
Description | Data deposited in or computed by PubChem | |
CAS No. |
12039-83-7 | |
Record name | Titanium silicide (TiSi2) | |
Source | CAS Common Chemistry | |
URL | https://commonchemistry.cas.org/detail?cas_rn=12039-83-7 | |
Description | CAS Common Chemistry is an open community resource for accessing chemical information. Nearly 500,000 chemical substances from CAS REGISTRY cover areas of community interest, including common and frequently regulated chemicals, and those relevant to high school and undergraduate chemistry classes. This chemical information, curated by our expert scientists, is provided in alignment with our mission as a division of the American Chemical Society. | |
Explanation | The data from CAS Common Chemistry is provided under a CC-BY-NC 4.0 license, unless otherwise stated. | |
Record name | Titanium silicide (TiSi2) | |
Source | ChemIDplus | |
URL | https://pubchem.ncbi.nlm.nih.gov/substance/?source=chemidplus&sourceid=0012039837 | |
Description | ChemIDplus is a free, web search system that provides access to the structure and nomenclature authority files used for the identification of chemical substances cited in National Library of Medicine (NLM) databases, including the TOXNET system. | |
Record name | Titanium silicide (TiSi2) | |
Source | EPA Chemicals under the TSCA | |
URL | https://www.epa.gov/chemicals-under-tsca | |
Description | EPA Chemicals under the Toxic Substances Control Act (TSCA) collection contains information on chemicals and their regulations under TSCA, including non-confidential content from the TSCA Chemical Substance Inventory and Chemical Data Reporting. | |
Record name | Titanium disilicide | |
Source | EPA DSSTox | |
URL | https://comptox.epa.gov/dashboard/DTXSID70894998 | |
Description | DSSTox provides a high quality public chemistry resource for supporting improved predictive toxicology. | |
Record name | Titanium disilicide | |
Source | European Chemicals Agency (ECHA) | |
URL | https://echa.europa.eu/substance-information/-/substanceinfo/100.031.719 | |
Description | The European Chemicals Agency (ECHA) is an agency of the European Union which is the driving force among regulatory authorities in implementing the EU's groundbreaking chemicals legislation for the benefit of human health and the environment as well as for innovation and competitiveness. | |
Explanation | Use of the information, documents and data from the ECHA website is subject to the terms and conditions of this Legal Notice, and subject to other binding limitations provided for under applicable law, the information, documents and data made available on the ECHA website may be reproduced, distributed and/or used, totally or in part, for non-commercial purposes provided that ECHA is acknowledged as the source: "Source: European Chemicals Agency, http://echa.europa.eu/". Such acknowledgement must be included in each copy of the material. ECHA permits and encourages organisations and individuals to create links to the ECHA website under the following cumulative conditions: Links can only be made to webpages that provide a link to the Legal Notice page. | |
Disclaimer and Information on In-Vitro Research Products
Please be aware that all articles and product information presented on BenchChem are intended solely for informational purposes. The products available for purchase on BenchChem are specifically designed for in-vitro studies, which are conducted outside of living organisms. In-vitro studies, derived from the Latin term "in glass," involve experiments performed in controlled laboratory settings using cells or tissues. It is important to note that these products are not categorized as medicines or drugs, and they have not received approval from the FDA for the prevention, treatment, or cure of any medical condition, ailment, or disease. We must emphasize that any form of bodily introduction of these products into humans or animals is strictly prohibited by law. It is essential to adhere to these guidelines to ensure compliance with legal and ethical standards in research and experimentation.