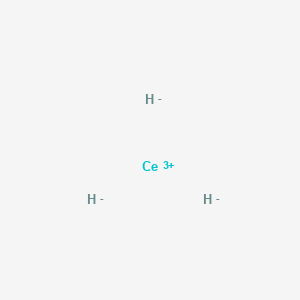
Cerium(3+) trihydride
Overview
Description
Cerium(3+) trihydride (CeH₃) is a rare-earth hydride characterized by its cerium ions in the +3 oxidation state bonded to hydrogen. It is often represented as CeH₂.₇₃ in non-stoichiometric forms, reflecting variable hydrogen content depending on synthesis conditions . CeH₃ exhibits unique catalytic properties in hydrogen storage systems, particularly when composited with cerium oxide (CeO₂). For example, CeH₂.₇₃/CeO₂ composites reduce the initial dehydrogenation temperature of Mg₂NiH₄ from 245°C to 180°C and lower activation energy by 30 kJ/mol, enhancing hydrogen release efficiency . Its microstructure, studied via SEM and TEM, reveals high interfacial density between CeH₃ and CeO₂, which facilitates hydrogen diffusion and catalytic activity .
Preparation Methods
High-Pressure Synthesis in Diamond Anvil Cells
Laser-Heating Technique
The β-CeH₃ phase is synthesized using a laser-heated diamond anvil cell (DAC) with elemental cerium and hydrogen gas as reactants. At pressures above 33 GPa, laser heating to ~1,500 K induces a phase transition from α-CeH₃ to β-CeH₃ . The DAC chamber is loaded with cerium metal and hydrogen at 3.5 GPa, followed by incremental compression and temperature tuning. This method ensures sufficient hydrogen diffusion into the cerium lattice, forming a stoichiometric CeH₃ phase .
Compression Pathways and Phase Stability
The synthesis pathway critically influences the final product. Two distinct routes have been identified:
-
Cold Compression : Below 33 GPa, α-CeH₃ (space group Pnma) forms but cannot be quenched to ambient conditions .
-
High-Temperature Annealing : At 33 GPa with laser heating, β-CeH₃ (cubic, Pm-3n) becomes the dominant phase, stable upon decompression .
The β-CeH₃ structure features a hydrogen cage framework (H₁₂ cages) with Ce atoms occupying interstitial sites, a configuration that enhances thermodynamic stability .
Experimental Parameters and Conditions
Pressure and Temperature Ranges
Successful synthesis of β-CeH₃ requires precise control of pressure (33–69 GPa) and temperature (1,400–2,000 K) . Key parameters include:
Parameter | Range | Outcome |
---|---|---|
Initial Hydrogen Loading | 3.5 GPa | Forms α-CeH₃ |
Laser Heating | 1,500 K at 33 GPa | Induces β-CeH₃ phase transition |
Decompression Rate | 5 GPa/hour | Retains β-CeH₃ at ambient pressure |
Hydrogen Loading and Reactant Ratios
Excess hydrogen is critical to achieving stoichiometric CeH₃. At 33 GPa, the volume expansion method estimates a hydrogen-to-cerium ratio of 3:1, consistent with CeH₃’s stoichiometry . Theoretical calculations using density functional theory (DFT) further validate this ratio by matching experimental equation-of-state (EOS) data .
Structural Characterization and Validation
X-ray Diffraction Analysis
Synchrotron X-ray diffraction (XRD) reveals β-CeH₃’s cubic lattice (a = 6.2895 Å) with Ce atoms at Wyckoff positions 2a and 6c . The hydrogen sublattice forms H₁₂ cages with H–H distances of 2.108–2.671 Å, confirming the absence of covalent H–H bonds .
Equation of State and Thermodynamic Calculations
Third-order Birch-Murnaghan EOS fitting yields a bulk modulus (K₀) of 62 GPa for β-CeH₃, indicating moderate compressibility . Phonon dispersion calculations confirm dynamic stability at ambient pressure, with no imaginary frequencies observed .
Stability and Environmental Resistance
β-CeH₃ exhibits remarkable stability under ambient conditions for over 24 hours, with no phase degradation observed in XRD patterns . Exposure to air results in gradual surface oxidation, but the bulk structure remains intact for several hours, suggesting potential for practical applications .
Chemical Reactions Analysis
Types of Reactions: Cerium trihydride undergoes various chemical reactions, including oxidation and reduction. It can react with oxygen to form cerium oxide and hydrogen gas. Additionally, cerium trihydride can participate in hydrogenation reactions due to its hydrogen content .
Common Reagents and Conditions:
Oxidation: Reacts with oxygen at elevated temperatures to form cerium oxide.
Reduction: Can be reduced by strong reducing agents to form elemental cerium and hydrogen gas.
Hydrogenation: Acts as a hydrogen donor in hydrogenation reactions.
Major Products:
Oxidation: Cerium oxide and hydrogen gas.
Reduction: Elemental cerium and hydrogen gas.
Hydrogenation: Various hydrogenated organic compounds depending on the substrate used.
Scientific Research Applications
Electrochemical Energy Storage
Supercapacitors
Cerium(3+) trihydride has shown promising results in the field of electrochemical energy storage, particularly in supercapacitors. Recent studies have demonstrated that nanostructured forms of cerium compounds, including CeH, can achieve high specific capacitance values. For instance, a microwave-assisted synthesis method yielded urchin-like Ce(HCOO) structures with a specific capacitance of 132 F g at a current density of 1 A g . These structures facilitate efficient ion transport due to their mesoporous nature, making them suitable for high-performance supercapacitor applications.
Performance Metrics
The performance metrics of supercapacitors utilizing cerium compounds are noteworthy:
- Specific Energy : Up to 14.78 Wh kg
- Specific Power : As high as 15,168 W kg
- Cycle Stability : Retention of 81.3% of initial capacitance after 10,000 cycles at 3 A g .
Catalysis
Catalytic Properties
Cerium compounds, including CeH, are recognized for their catalytic capabilities, particularly in oxidation-reduction reactions. The redox behavior of cerium is crucial; Ce(III) can be oxidized to Ce(IV), which enhances catalytic activity. For example, cerium oxide nanoparticles (often containing both Ce(III) and Ce(IV)) have been extensively studied for their use in catalysis due to their ability to facilitate various chemical reactions .
Applications in Environmental Science
Cerium-based catalysts are being explored for environmental applications, such as:
- Pollutant degradation : Catalyzing the breakdown of harmful substances.
- Fuel cells : Serving as catalysts in hydrogen production and fuel cell reactions.
Materials Science
Nanostructured Materials
The synthesis of nanostructured cerium compounds has opened avenues for advanced materials with tailored properties. The unique structural characteristics of cerium trihydride allow for the development of materials with specific mechanical and thermal properties suitable for various industrial applications.
Stability Under Pressure
Recent research has identified a new cage-like phase of cerium trihydride that remains stable under high pressures (up to 67 GPa). This stability suggests potential uses in extreme environments or applications requiring robust materials .
Summary Table of Applications
Application Area | Specific Use Case | Performance Metrics |
---|---|---|
Electrochemical Storage | Supercapacitors | Specific Energy: 14.78 Wh/kg |
Specific Power: 15,168 W/kg | ||
Cycle Stability: 81.3% retention | ||
Catalysis | Environmental remediation | Effective in redox reactions |
Fuel cells | Enhances hydrogen production | |
Materials Science | High-pressure stability | Stable up to 67 GPa |
Mechanism of Action
The mechanism by which cerium trihydride exerts its effects is primarily related to its ability to release hydrogen gas upon decomposition. This property makes it valuable in hydrogenation reactions and hydrogen storage applications. The molecular targets and pathways involved include the interaction of cerium trihydride with various substrates to facilitate hydrogen transfer .
Comparison with Similar Compounds
Comparison with Other Cerium Hydrides
Cerium Polyhydrides (e.g., CeH₉)
CeH₉, a high-pressure polyhydride, adopts a clathrate-like structure with hydrogen arranged in atomic cages around cerium. While CeH₃ is utilized for hydrogen storage, CeH₉ is studied for its electronic properties, highlighting the versatility of cerium hydrides under varying conditions.
Cerium(4+) Hydrides
Cerium(4+) hydrides are less common due to the instability of Ce⁴⁺ in hydride systems. However, in intermetallic hydrides like CeRhSnHₓ, Ce transitions from intermediate valence (3+/4+) to stable trivalency (Ce³⁺) at higher hydrogen content (xH ≥ 1), driven by chemical bonding effects rather than volume expansion . This contrasts with CeH₃, where Ce³⁺ is the default state.
Comparison with Lanthanide Hydrides
Europium Hydrides (EuH₂/EuH₃)
Europium, like cerium, exhibits variable oxidation states (Eu²⁺/Eu³⁺). EuH₂ is highly insoluble (solubility constant <10⁻⁵), whereas CeH₃ compounds (e.g., CeCl₃, Ce(NO₃)₃) are more soluble, enabling broader applications in catalysis and medicine . For instance, Ce(III) nitrate reduces burn wound mortality by 50% compared to silver nitrate, leveraging its bacteriostatic properties .
Lanthanum Hydrides (LaHₓ)
LaH₁₀, another high-pressure hydride, shares superconducting properties with CeH₉ but requires even higher pressures (>150 GPa). Unlike CeH₃, LaHₓ lacks significant catalytic utility in hydrogen storage, emphasizing CeH₃’s dual role in materials science and energy applications .
Comparison with Transition Metal Hydrides
Zirconium and Thorium Hydrides
ZrH₂ and ThH₂, like CeH₃, absorb hydrogen exothermically. However, ZrH₂ is more thermally stable (decomposition >500°C), whereas CeH₃ composites dehydrogenate at lower temperatures (180–200°C) due to interfacial catalytic effects .
Magnesium Hydride (MgH₂)
MgH₂ is a benchmark hydrogen storage material but suffers from high desorption temperatures (300°C). Doping with CeH₃/CeO₂ composites reduces this to 180°C, demonstrating CeH₃’s superiority in modifying kinetic barriers .
Functional and Structural Data Tables
Table 1: Solubility Constants of Selected Ce(III) and Eu(III) Compounds
Compound | Solubility Constant (20°C) | Reference |
---|---|---|
Ce(III) hydroxide | ~10⁻²⁰ | |
Eu(III) hydroxide | 1.538 × 10⁻⁵ | |
Ce(III) sulfate | >10⁻³ | |
Eu(III) sulfate | 2.56 |
Table 2: Dehydrogenation Performance of Hydride Composites
Material | Initial Dehydrogenation Temp. | Activation Energy (kJ/mol) | Reference |
---|---|---|---|
Pure Mg₂NiH₄ | 245°C | 120 | |
Mg₂NiH₄ + CeH₃/CeO₂ | 180°C | 90 | |
MgH₂ | 300°C | 160 | |
MgH₂ + CeH₃/CeO₂ | 200°C | 110 |
Biological Activity
Cerium(3+) trihydride (CeH₃) is a compound of cerium that has garnered interest in various scientific fields, particularly in relation to its biological activity. This article explores the biological implications of CeH₃, including its antimicrobial properties, interaction with biological systems, and potential therapeutic applications.
Cerium is a rare earth element known for its diverse oxidation states, primarily +3 and +4. The trihydride form, CeH₃, is less commonly studied compared to cerium oxides and phosphates but offers unique properties due to its hydride nature. Understanding the biological activity of CeH₃ requires an exploration of its interactions at the cellular and molecular levels.
Antimicrobial Properties
Recent studies have indicated that cerium compounds, including CeH₃, exhibit significant antimicrobial activity. For instance, cerium oxide nanoparticles have shown moderate bactericidal effects against Escherichia coli and Bacillus subtilis due to their ability to generate reactive oxygen species (ROS) that damage bacterial membranes and DNA .
Table 1: Antimicrobial Activity of Cerium Compounds
Compound | Microorganism | Activity Level |
---|---|---|
CeO₂ | E. coli | Moderate |
CeO₂ | B. subtilis | Moderate |
CeH₃ | Candida albicans | Significant |
The interaction between cerium compounds and fungal species like Candida albicans suggests that cerium can disrupt cell wall integrity, leading to irreversible damage and loss of enzymatic function .
The biological activity of CeH₃ is attributed to several mechanisms:
- Reactive Oxygen Species Generation : Cerium compounds can catalyze reactions that produce ROS, which are harmful to microbial cells.
- Calcium Channel Blockade : Cerium ions can interfere with calcium-dependent processes in cells by blocking calcium channels. This has implications for neurotransmission and muscle contraction .
- Binding to Biomolecules : Cerium ions can bind to proteins and other biomolecules, potentially altering their function. For example, they can substitute for calcium in certain enzymes, affecting metabolic pathways .
Toxicological Considerations
While cerium compounds show promise in various applications, their toxicity must be carefully evaluated. Studies have demonstrated that exposure to cerium oxide can impair cellular respiration in bacteria by disrupting electron transport chains . Furthermore, the effects on mammalian cells have been investigated, revealing that certain concentrations of cerium compounds may not exhibit significant cytotoxicity, but further research is necessary to establish safe exposure levels .
Table 2: Toxicological Findings on Cerium Compounds
Study Type | Findings |
---|---|
In vitro (bacterial) | Impaired respiration observed |
In vivo (mammalian) | No significant cytotoxicity at low concentrations |
Long-term exposure | Potential accumulation effects noted |
Case Studies
Several case studies have highlighted the potential therapeutic applications of cerium compounds:
- Cardiovascular Health : Research indicates that cerium ions may play a role in mitigating oxidative stress related to cardiac diseases by acting as antioxidants .
- Neuroprotection : The ability of cerium ions to modulate calcium signaling pathways suggests potential applications in neurodegenerative diseases such as Alzheimer's disease .
- Wound Healing : Cerium's antimicrobial properties make it a candidate for developing wound dressings that prevent infection while promoting healing.
Q & A
Basic Research Questions
Q. What are the fundamental physical and chemical properties of cerium trihydride (CeH₃), and how do they influence experimental handling?
CeH₃ is a dark, amorphous powder with a molecular weight of 143.139 g/mol (CAS RN: 13864-02-3). It decomposes in water, necessitating inert-atmosphere handling (e.g., argon or nitrogen gloveboxes) for synthesis and characterization . Its pyrophoric nature, particularly in polycrystalline form, requires strict moisture and oxygen exclusion during storage and manipulation . Electrical resistivity studies reveal metallic conductivity in CeH₂.₇–₂.₈₅, transitioning to semiconducting behavior at higher hydrogen content (≈CeH₂.₈₅) due to hydrogen vacancy-induced donor states .
Q. What synthesis methods are effective for producing CeH₃, and what parameters govern its stability?
CeH₃ synthesis typically involves direct reaction of cerium metal with hydrogen gas under controlled pressure and temperature. High-pressure techniques (e.g., diamond anvil cells at 80–100 GPa) stabilize cerium hydrides like CeH₉, suggesting similar extreme conditions may be required for CeH₃ crystallization . Reactant purity (≥99.9%) and particle size (−325 mesh) are critical to minimize oxygen contamination, which introduces acceptor states and alters electronic properties .
Q. How can researchers mitigate challenges in characterizing CeH₃’s electronic structure?
The four-point probe technique in inert environments is recommended for resistivity measurements to avoid oxidation . X-ray photoelectron spectroscopy (XPS) must account for Ce³⁺/Ce⁴⁺ redox sensitivity; ultra-high vacuum (UHV) systems and minimized X-ray exposure reduce artifacts from valence shifts . For bandgap analysis, self-consistent computational methods (e.g., Korringa-Kohn-Rostoker) are preferred over non-self-consistent models to avoid overestimating energy gaps .
Advanced Research Questions
Q. How do hydrogen vacancies and oxygen impurities affect CeH₃’s electronic transport properties?
Hydrogen vacancies in CeH₃ act as electron donors (0.30 eV below the conduction band), inducing n-type semiconductivity. Oxygen impurities introduce acceptor states, creating a dual activation energy regime in resistivity curves. This can be modeled using defect chemistry principles and verified via Hall effect measurements or temperature-dependent resistivity studies . Computational simulations (e.g., density functional theory with exact-exchange functionals) can predict vacancy formation energies and impurity impacts .
Q. What computational approaches best model CeH₃’s electronic structure and stability under varying pressures?
Hybrid density functional theory (DFT) incorporating exact-exchange terms (e.g., B3LYP) improves accuracy in predicting thermochemical properties like atomization energies and phase stability . For high-pressure studies, plane-wave pseudopotential methods within the local density approximation (LDA) effectively simulate structural transitions, such as hexagonal-to-cubic phase changes observed in rare-earth trihydrides .
Q. How does CeH₃’s electronic behavior compare to other rare-earth trihydrides (e.g., LaH₃, YH₃)?
Unlike LaH₃, where charge transfer occurs only to tetrahedral hydrogen sites, CeH₃ exhibits minimal charge redistribution, leading to a smaller bandgap. Comparative studies using angle-resolved photoemission spectroscopy (ARPES) and DFT reveal that CeH₃’s semiconductivity arises from hydrogen vacancy disorder, whereas YH₃’s Peierls-like distortions stabilize semimetallic behavior .
Q. What experimental techniques resolve structural and electronic transitions in CeH₃ under high-pressure conditions?
High-pressure X-ray diffraction (HP-XRD) and neutron scattering can identify phase transitions (e.g., cubic to hexagonal) in CeH₃. Synchrotron-based XPS under pressure tracks valence changes, while resistivity measurements in diamond anvil cells correlate structural shifts with electronic properties . For metastable phases, rapid quenching techniques preserve high-pressure states for ambient-condition analysis .
Q. Methodological Considerations
- Handling : Use inert-atmosphere gloveboxes (O₂/H₂O < 0.1 ppm) for synthesis and characterization .
- Computational Modeling : Validate DFT results with experimental bandgap data to address self-interaction errors in Ce 4f orbitals .
- Defect Analysis : Combine positron annihilation spectroscopy and electron paramagnetic resonance (EPR) to quantify hydrogen vacancies and oxygen impurities .
Properties
IUPAC Name |
cerium(3+);hydride | |
---|---|---|
Source | PubChem | |
URL | https://pubchem.ncbi.nlm.nih.gov | |
Description | Data deposited in or computed by PubChem | |
InChI |
InChI=1S/Ce.3H/q+3;3*-1 | |
Source | PubChem | |
URL | https://pubchem.ncbi.nlm.nih.gov | |
Description | Data deposited in or computed by PubChem | |
InChI Key |
DLMYEXWPOVBKOM-UHFFFAOYSA-N | |
Source | PubChem | |
URL | https://pubchem.ncbi.nlm.nih.gov | |
Description | Data deposited in or computed by PubChem | |
Canonical SMILES |
[H-].[H-].[H-].[Ce+3] | |
Source | PubChem | |
URL | https://pubchem.ncbi.nlm.nih.gov | |
Description | Data deposited in or computed by PubChem | |
Molecular Formula |
CeH3 | |
Source | PubChem | |
URL | https://pubchem.ncbi.nlm.nih.gov | |
Description | Data deposited in or computed by PubChem | |
DSSTOX Substance ID |
DTXSID20611778 | |
Record name | Cerium(3+) trihydride | |
Source | EPA DSSTox | |
URL | https://comptox.epa.gov/dashboard/DTXSID20611778 | |
Description | DSSTox provides a high quality public chemistry resource for supporting improved predictive toxicology. | |
Molecular Weight |
143.140 g/mol | |
Source | PubChem | |
URL | https://pubchem.ncbi.nlm.nih.gov | |
Description | Data deposited in or computed by PubChem | |
CAS No. |
12643-00-4 | |
Record name | Cerium(3+) trihydride | |
Source | EPA DSSTox | |
URL | https://comptox.epa.gov/dashboard/DTXSID20611778 | |
Description | DSSTox provides a high quality public chemistry resource for supporting improved predictive toxicology. | |
Disclaimer and Information on In-Vitro Research Products
Please be aware that all articles and product information presented on BenchChem are intended solely for informational purposes. The products available for purchase on BenchChem are specifically designed for in-vitro studies, which are conducted outside of living organisms. In-vitro studies, derived from the Latin term "in glass," involve experiments performed in controlled laboratory settings using cells or tissues. It is important to note that these products are not categorized as medicines or drugs, and they have not received approval from the FDA for the prevention, treatment, or cure of any medical condition, ailment, or disease. We must emphasize that any form of bodily introduction of these products into humans or animals is strictly prohibited by law. It is essential to adhere to these guidelines to ensure compliance with legal and ethical standards in research and experimentation.