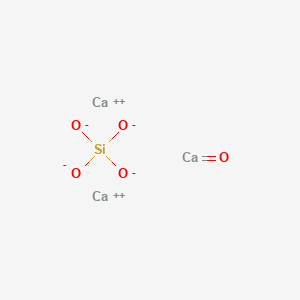
Tricalcium silicate
Overview
Description
Tricalcium silicate (C₃S), also known as alite, is the primary constituent of Portland cement clinker, accounting for 50–70% of its composition. It is a key driver of cement hydration, contributing to early and long-term strength development through the formation of calcium silicate hydrate (C-S-H) gels and calcium hydroxide . C₃S exists in seven polymorphic forms: three triclinic (T1, T2, T3), three monoclinic (M1, M2, M3), and one rhombohedral (R), with structural variations influencing reactivity .
In biomedical applications, C₃S is a cornerstone of hydraulic calcium silicate cements (e.g., Mineral Trioxide Aggregate, MTA), valued for its osteoconductivity, self-setting properties, and biocompatibility . Recent advancements include doping with strontium (Sr) or iron (Fe) to enhance mechanical performance and reduce alkalinity, addressing limitations like high pH (~12) and slow degradation .
Preparation Methods
Solid-State Reaction Synthesis
Procedure and Parameters
The conventional solid-state reaction involves mixing stoichiometric amounts of calcium carbonate (CaCO₃) and silicon dioxide (SiO₂) in a 3:1 molar ratio, often with additives like boric acid (0.5 wt%) to lower the synthesis temperature . The blended powders are pre-pressed into pellets and subjected to multi-stage calcination: initially at 1,000°C for 2 hours to decompose CaCO₃ into CaO, followed by high-temperature sintering at 1,450°C for 2 hours . This prolonged thermal treatment ensures complete reaction but risks partial decomposition of this compound into dicalcium silicate (Ca₂SiO₄) and free lime (CaO) if cooling rates are insufficient .
Advantages and Limitations
Solid-state synthesis is industrially favored for its scalability and minimal reagent complexity. However, it necessitates energy-intensive conditions (>1,400°C) and prolonged sintering times (6–12 hours), often yielding coarse particles (10–50 µm) with residual CaO impurities . The presence of free lime can compromise hydraulic reactivity and long-term stability in cementitious systems .
Sol-Gel Method
Procedure and Parameters
The sol-gel technique employs calcium nitrate tetrahydrate (Ca(NO₃)₂·4H₂O) and tetraethyl orthosilicate (TEOS) as precursors, dissolved in aqueous solution at pH 4.5 . After gelation at 50–75°C, the dried gel is calcined at 1,500°C for 9 hours, producing nano-sized this compound particles (20–50 nm) . This method achieves molecular-level homogeneity, reducing the need for repeated grinding and sintering cycles compared to solid-state reactions.
Advantages and Limitations
Sol-gel-derived this compound exhibits superior hydration kinetics due to its high surface area (15–30 m²/g) and nanoscale morphology . However, the reliance on expensive alkoxide precursors and precise pH control increases production costs, limiting large-scale applicability. Additionally, residual nitrate groups from incomplete combustion may introduce impurities .
Pechini Method
Procedure and Parameters
The Pechini method, a polymeric precursor route, combines Ca(NO₃)₂·4H₂O and colloidal SiO₂ with citric acid (CA) and ethylene glycol (EG) in a CA:EG molar ratio of 1:2 . The chelated resin is calcined at 1,200–1,400°C for 3 hours, yielding phase-pure this compound with minimal free CaO content . This method circumvents the need for mechanical mixing, ensuring atomic-scale stoichiometric control.
Advantages and Limitations
The Pechini technique achieves high purity (>95% Ca₃SiO₅) at lower temperatures (1,200°C vs. 1,450°C for solid-state) . However, extended calcination times (24–72 hours) are required to eliminate intermediate phases like Ca₁₂Al₁₄O₃₃ . The method’s dependency on organic reagents also complicates waste management.
Microwave-Assisted Sintering
Procedure and Parameters
A recent patent discloses a hybrid microwave approach using auxiliary heating bodies (e.g., SiC) to sinter CaCO₃-SiO₂ compacts at 1,200–1,500°C for 0.5–1.5 hours . Microwave radiation enhances heat transfer, enabling rapid densification and inhibiting CaO formation through uniform thermal gradients .
Advantages and Limitations
This method reduces energy consumption by 40–50% compared to conventional furnaces and achieves >90% phase purity in under 2 hours . However, the requirement for microwave-compatible susceptors and specialized equipment poses scalability challenges.
Comparative Analysis of Preparation Methods
Table 1: Synthesis Parameters and Outcomes
Table 2: Hydration and Mechanical Properties
Method | Setting Time (min) | Compressive Strength (MPa) | Porosity (%) |
---|---|---|---|
Solid-State | 120–180 | 5–7 | 55–60 |
Sol-Gel | 45–60 | 8–10 | 50–55 |
Pechini | 90–120 | 7–9 | 58–62 |
Microwave | 60–90 | 6–8 | 60–65 |
Impact of Synthesis Parameters on Material Properties
Particle size and morphology directly influence this compound’s hydraulic reactivity. Nano-sized sol-gel particles accelerate early-age hydration by providing nucleation sites for calcium silicate hydrate (C-S-H) gel . Conversely, microwave-sintered materials exhibit interconnected pore networks (60–65% porosity), enhancing permeability in biomedical scaffolds . The Pechini method’s stoichiometric precision minimizes deleterious phases, improving long-term compressive strength .
Chemical Reactions Analysis
Hydration Reactions
Tricalcium silicate (Ca₃SiO₅, C₃S) undergoes hydration to form calcium silicate hydrate (C-S-H) and calcium hydroxide (Ca(OH)₂), which are critical for cement hardening. The reaction is exothermic and proceeds in multiple stages :
Primary Reaction:
Key Observations:
-
Stage 1 (Initial Dissolution): Rapid release of Ca²⁺ and OH⁻ ions raises the pH to >12 .
-
Stage 2 (Dormant Period): Reaction slows as ions saturate the solution .
-
Stage 3 (Acceleration): Nucleation and growth of C-S-H and Ca(OH)₂ crystals dominate, driven by diffusion through the C-S-H layer .
Factors Influencing Hydration:
Carbonation Reactions
Under high CO₂ concentrations, C₃S reacts to form calcium carbonate (CaCO₃) and silica gel (SiO₂) :
Reaction Pathway:
Research Findings:
-
Early Carbonation (Gas Phase): Produces low-lime C-S-H and calcite. Reaction rate peaks at intermediate CO₂ pressures .
-
Late Carbonation (Liquid Phase): Forms polymerized silica and aragonite, with rate dependent on hydration barrier fragmentation .
Kinetic Behavior:
Carbonation Phase | Rate Trend | Dominant Products |
---|---|---|
Gas Phase | Increases then decreases | Calcite, Amorphous SiO₂ |
Liquid Phase | Decreases then increases | Aragonite, Polymerized SiO₂ |
Thermal Decomposition
At high temperatures (1,000–1,300°C), C₃S decomposes into dicalcium silicate (Ca₂SiO₄) and free lime (CaO) :
Reaction:
Experimental Data:
Temperature (°C) | Decomposition Rate (Free CaO after 24 hrs) |
---|---|
1,000 | 0.2% |
1,175 | 3.0% (Maximum) |
1,300 | Negligible |
Decomposition is autocatalytic, accelerated by pre-existing CaO or Ca₂SiO₄ .
Formation from Raw Materials
Industrial synthesis involves heating limestone (CaCO₃) and silica (SiO₂) at ~1,450°C :
Synthetic Methods:
-
Pechini Technique: Uses calcium nitrate and tetraethyl orthosilicate precursors, calcined at 1,300°C.
-
Spark Plasma Sintering: Produces high-density C₃S with superior mechanical properties .
Reaction Kinetics and Mechanisms
Hydration Control Factors:
-
Nucleation Density: Higher C-S-H nucleation sites accelerate hydration .
-
Ion Diffusion: Governed by C-S-H layer thickness, which limits water access .
Advanced Characterization:
Scientific Research Applications
Construction Materials
1.1. Role in Cement and Concrete
Tricalcium silicate is a primary component of Portland cement, where it contributes significantly to the hydration process and strength development of concrete. Upon hydration, it reacts to form calcium silicate hydrate (C-S-H) gel and calcium hydroxide, which are essential for the mechanical properties of concrete. The hydration process can be summarized as follows:
The formation of C-S-H gel provides the binding matrix that gives concrete its strength and durability. Studies have shown that optimizing the particle size and distribution of this compound can enhance the performance of engineered cementitious composites (ECC) by improving ductility and resistance to cracking .
1.2. Engineered Cementitious Composites
Recent research indicates that this compound can be utilized in engineered cementitious composites to improve their mechanical properties under extreme conditions. This includes enhancing tensile strain capacity and resilience against environmental hazards . The incorporation of this compound in ECC formulations has demonstrated significant improvements in both flexural strength and ductility when combined with various mineral additives .
Biomedical Applications
2.1. Dental Materials
This compound-based cements have gained prominence in dental applications due to their excellent biocompatibility and ability to promote biomineralization. These materials are used for root canal obturation and as reparative agents in endodontics. Research shows that this compound cements exhibit superior sealing properties compared to traditional materials, facilitating better healing outcomes .
- Case Study: Biodentine™
Biodentine™, a commercially available this compound-based material, has been shown to support stem cell differentiation and exhibit minimal cytotoxic effects when tested against human dental pulp cells . Its ability to release calcium ions promotes mineralization, making it an effective choice for pulp capping procedures.
2.2. Antibacterial Properties
This compound cements also possess antibacterial properties, which are crucial for preventing infections during dental procedures. Studies have indicated that these materials can inhibit biofilm formation by bacteria such as Enterococcus faecalis, a common pathogen in endodontic failures . The high pH generated during hydration contributes to this antimicrobial effect.
Environmental Considerations
3.1. Sustainable Construction Practices
The use of this compound in construction materials aligns with sustainable practices by reducing the carbon footprint associated with traditional Portland cement production. Innovations in synthesizing this compound from industrial byproducts or alternative raw materials are being explored to minimize environmental impact while maintaining performance standards .
3.2. Waste Management
This compound's ability to bind heavy metals and other pollutants makes it a candidate for use in waste management applications, particularly in stabilizing contaminated soils or sediments . Its chemical properties allow for effective immobilization of hazardous substances, thereby preventing leaching into groundwater.
Mechanism of Action
The mechanism of action of tricalcium silicate involves its hydration reaction with water. Upon mixing with water, this compound dissolves and reacts to form calcium silicate hydrate and calcium hydroxide. The calcium silicate hydrate forms a gel-like structure that binds the cement particles together, providing strength and durability. The calcium hydroxide contributes to the alkalinity of the cement paste, which helps in protecting the embedded steel reinforcement from corrosion .
Comparison with Similar Compounds
Comparison with Tricalcium Aluminate (C₃A)
Chemical Structure and Reactivity
- C₃S : Ca₃SiO₅; slower hydration kinetics, forming C-S-H and portlandite (Ca(OH)₂) .
- C₃A : Ca₃Al₂O₆; rapid hydration, forming ettringite (C₆AŠ₃H₃₂) in the presence of gypsum .
Hydration Behavior
Property | C₃S | C₃A |
---|---|---|
Initial Hydration Rate | Moderate | Very Fast |
Key Products | C-S-H, Ca(OH)₂ | Ettringite, C-A-S-H |
Heat Release | Sustained over days | Intense, early-stage |
C₃A’s rapid reaction necessitates gypsum addition to prevent flash setting, whereas C₃S governs long-term strength .
Comparison with Dicalcium Silicate (C₂S)
Hydration and Strength Development
- C₃S : High early strength (70% within 28 days); dominant in Portland cement .
- C₂S (Belite) : Ca₂SiO₄; slower hydration, contributing to later strength (post-28 days) .
Research Findings
- C₃S : Forms C-S-H with higher Ca/Si ratio (~1.7) compared to C₂S-derived C-S-H (~1.5) .
- C₂S : Lower heat release, making it suitable for mass concrete applications .
Comparison with Tetracalcium Aluminoferrite (C₄AF)
Role in Cement Systems
- C₃S : Primary strength contributor; minimal iron content.
- C₄AF : Ca₄Al₂Fe₂O₁₀; contributes to cement color and moderates hydration heat.
Key Differences
Property | C₃S | C₄AF |
---|---|---|
Reactivity | High | Moderate |
Hydration Products | C-S-H, Ca(OH)₂ | Ferrite-rich C-A-S-H |
Iron Content | None | Up to 15% in clinker |
C₄AF enhances durability in sulfate-rich environments but offers lower mechanical strength than C₃S .
Comparison with Hydroxyapatite (HAp) Composites
Performance Metrics
Property | C₃S | C₃S/HAp Composite |
---|---|---|
Compressive Strength | 20 MPa | 35 MPa |
Water Absorption | High | Suppressed by HAp |
Biocompatibility | Moderate (high pH) | Enhanced (lower pH, Sr doping) |
HAp addition enhances bioactivity, mimicking natural bone mineralogy .
Comparison with Doped Tricalcium Silicate Formulations
Iron-Doped vs. Strontium-Doped C₃S
Property | Fe-doped C₃S (5 mol% Fe) | Sr-doped C₃S (1.75% Sr) |
---|---|---|
pH | 8.80 | ~10.5 |
Porosity | 45% | 30% |
Bioactivity | Enhanced HA formation | Improved osteogenesis |
Iron doping reduces alkalinity and increases porosity, favoring cell adhesion, while Sr promotes bone regeneration .
Comparison with Other Calcium Silicate-Based Biomaterials
Dental Materials: C₃S vs. Dicalcium Silicate (C₂S) Sealers
- C₃S Sealers : 97.3% success rate in pulpotomies; superior to formocresol (91.4%) .
- C₂S Sealers : Comparable biocompatibility but slower setting and lower early strength .
Hydraulic Conductivity
- C₃S : Lower hydraulic conductivity than C₂S due to denser C-S-H structure .
Biological Activity
Tricalcium silicate (C3S) is a key component in various biomedical applications, particularly in dentistry and bone regeneration. Its biological activity is characterized by its ability to promote cellular responses, biomineralization, and tissue regeneration. This article explores the biological effects of this compound, focusing on its applications in dental materials, osteogenic differentiation, and its physicochemical properties.
Overview of this compound
This compound is a silicate mineral that plays a crucial role in the formulation of various bioceramics, including mineral trioxide aggregate (MTA) and Biodentine. These materials are widely used for their biocompatibility and ability to induce mineralization in dental and bone tissues.
1. Cell Viability and Cytotoxicity
Research indicates that the cytotoxicity of this compound-based materials can vary depending on the setting conditions. A study on Biodentine, a this compound-containing cement, revealed that extracts taken during the setting process exhibited cytotoxic effects on stem cells from human exfoliated deciduous teeth (SHED). In contrast, extracts taken after setting showed no significant cytotoxicity, emphasizing the importance of timing in clinical applications .
2. Osteogenic Differentiation
This compound has been shown to influence osteogenic differentiation in human bone marrow-derived mesenchymal stem cells (hBM-MSCs). A study assessed the effects of various this compound cements on osteogenic markers such as alkaline phosphatase (ALP) and osteocalcin (OCN). Results indicated that while there was downregulation of certain osteogenic markers over time, this compound did not significantly enhance osteogenic differentiation compared to untreated controls . This suggests that while C3S has bioactive properties, its effectiveness may depend on specific formulations or conditions.
The physicochemical characteristics of this compound contribute significantly to its biological activity. Key properties include:
- pH Levels : this compound exhibits high pH levels upon hydration, which is associated with its antimicrobial effects. The alkalinization of the medium can inhibit bacterial growth, making it suitable for dental applications .
- Calcium Ion Release : The release of calcium ions from this compound promotes biomineralization and enhances interactions with surrounding tissues .
- Antibiofilm Activity : Studies have shown that modified this compound cements demonstrate effective antibiofilm properties against common oral pathogens like Streptococcus mutans, which is critical for preventing secondary infections in dental applications .
Case Study 1: Biodentine Application
A clinical study evaluated the use of Biodentine in vital pulp therapy. The results indicated that Biodentine not only provided a suitable sealing ability but also promoted reparative dentin formation due to its bioactive properties. Patients treated with Biodentine showed favorable outcomes with minimal complications .
Case Study 2: Root Canal Sealing
In a systematic review assessing the sealing ability of this compound-based sealers in endodontics, it was found that these materials exhibited superior sealing capabilities compared to traditional materials. The presence of calcium phosphate facilitated better integration with dentinal tubules and promoted biomineralization processes .
Comparative Data Table
Property | This compound | Biodentine | MTA |
---|---|---|---|
Cytotoxicity | Low (after setting) | Low | Moderate |
pH Level | High | High | Moderate |
Calcium Ion Release | High | High | Moderate |
Antibiofilm Activity | Yes | Yes | Limited |
Osteogenic Differentiation | Limited | Moderate | Moderate |
Q & A
Basic Research Questions
Q. How should researchers design experiments to assess tricalcium silicate’s role in cement hydration kinetics?
- Methodology : Use multivariate regression models to isolate the contribution of this compound (C₃S) to heat emission (response variable). For example, in cement studies, predictors include C₃S (x₂), C₃A (x₁), and other clinker phases. Apply stepwise regression with α-entry (αE) and α-removal (αR) thresholds (e.g., α = 0.15) while mandating the inclusion of C₃S in all models to quantify its significance .
- Data Example : A regression output might reveal that C₃S contributes 45–60% of total heat emission, with collinearity-adjusted coefficients (e.g., β = 0.52 for x₂) .
Q. What methods are recommended for synthesizing high-purity this compound in laboratory settings?
- Methodology : Solid-state synthesis at 1,500°C using stoichiometric mixtures of CaCO₃ and SiO₂, followed by quenching to preserve polymorphic purity. Characterization via X-ray diffraction (XRD) and Raman spectroscopy ensures phase identification. For nano-crystalline C₃S, sol-gel methods with controlled calcination reduce impurity phases like free lime .
- Key Findings : Trace Al³⁺ or Mg²⁺ dopants can stabilize reactive polymorphs (e.g., monoclinic M3) during synthesis .
Q. Which in vitro assays are validated for evaluating this compound’s cytocompatibility in biomedical applications?
- Methodology : Use 3D cell cultures (e.g., dental pulp stem cells) to simulate direct material-tissue interaction. Assess viability via MTT assays and differentiation via alkaline phosphatase (ALP) activity. Compare results against controls like mineral trioxide aggregate (MTA) .
- Data Example : this compound (e.g., Biodentine™) shows >90% cell viability at 72 hours, with ALP activity 20% lower than MTA but comparable osteogenic gene expression (e.g., RUNX2, COL1A1) .
Advanced Research Questions
Q. How can synchrotron XRD and density functional theory (DFT) resolve contradictions in this compound’s polymorphism data?
- Methodology : Combine high-temperature synchrotron XRD (up to 1,070°C) to track phase transitions (e.g., trigonal → triclinic) with DFT calculations to model electronic structures. For example, DFT can quantify the energy differences (ΔE ≈ 2–5 kJ/mol) between polymorphs (e.g., T1 vs. M1), explaining stability under varying conditions .
- Conflict Resolution : Discrepancies in reported polymorph counts (e.g., 7 vs. 5 phases) arise from subtle superstructural variations detectable via Rietveld refinement .
Q. What molecular simulation approaches elucidate this compound’s anisotropic thermal expansion and hydration mechanisms?
- Methodology : Employ reactive force fields (ReaxFF) in molecular dynamics (MD) simulations to model Ca²⁺ and SiO₄⁴⁻ interactions during heating. Analyze thermal expansion coefficients (e.g., α_z = 12.5 × 10⁻⁶ K⁻¹ for the c-axis) and compare with experimental dilatometry data .
- Key Insight : Simulations reveal that Ca-O bond distortion in SiO₄ tetrahedra drives anisotropic expansion, affecting early-age cement shrinkage .
Q. How can researchers address discrepancies in hydration kinetics data between this compound polymorphs?
- Methodology : Use isothermal calorimetry paired with solid-state NMR (²⁹Si, ²⁷Al) to track intermediate phases (e.g., C-S-H nucleation). For example, M3 polymorphs hydrate 30% faster than T1 due to higher surface reactivity, confirmed by QENS (quasi-elastic neutron scattering) .
- Data Contradiction : Some studies report a dormant hydration phase, while others observe continuous reaction—this depends on particle size distribution and Al³⁺ contamination .
Q. Methodological Resources
Properties
IUPAC Name |
dicalcium;oxocalcium;silicate | |
---|---|---|
Source | PubChem | |
URL | https://pubchem.ncbi.nlm.nih.gov | |
Description | Data deposited in or computed by PubChem | |
InChI |
InChI=1S/3Ca.O4Si.O/c;;;1-5(2,3)4;/q;2*+2;-4; | |
Source | PubChem | |
URL | https://pubchem.ncbi.nlm.nih.gov | |
Description | Data deposited in or computed by PubChem | |
InChI Key |
BCAARMUWIRURQS-UHFFFAOYSA-N | |
Source | PubChem | |
URL | https://pubchem.ncbi.nlm.nih.gov | |
Description | Data deposited in or computed by PubChem | |
Canonical SMILES |
[O-][Si]([O-])([O-])[O-].O=[Ca].[Ca+2].[Ca+2] | |
Source | PubChem | |
URL | https://pubchem.ncbi.nlm.nih.gov | |
Description | Data deposited in or computed by PubChem | |
Molecular Formula |
Ca3O5Si | |
Source | PubChem | |
URL | https://pubchem.ncbi.nlm.nih.gov | |
Description | Data deposited in or computed by PubChem | |
DSSTOX Substance ID |
DTXSID6049804 | |
Record name | Tricalcium silicate | |
Source | EPA DSSTox | |
URL | https://comptox.epa.gov/dashboard/DTXSID6049804 | |
Description | DSSTox provides a high quality public chemistry resource for supporting improved predictive toxicology. | |
Molecular Weight |
228.32 g/mol | |
Source | PubChem | |
URL | https://pubchem.ncbi.nlm.nih.gov | |
Description | Data deposited in or computed by PubChem | |
Physical Description |
Dry Powder; Other Solid; Pellets or Large Crystals, Colorless solid; [HSDB] | |
Record name | Calcium oxide silicate (Ca3O(SiO4)) | |
Source | EPA Chemicals under the TSCA | |
URL | https://www.epa.gov/chemicals-under-tsca | |
Description | EPA Chemicals under the Toxic Substances Control Act (TSCA) collection contains information on chemicals and their regulations under TSCA, including non-confidential content from the TSCA Chemical Substance Inventory and Chemical Data Reporting. | |
Record name | Tricalcium silicate | |
Source | Haz-Map, Information on Hazardous Chemicals and Occupational Diseases | |
URL | https://haz-map.com/Agents/7520 | |
Description | Haz-Map® is an occupational health database designed for health and safety professionals and for consumers seeking information about the adverse effects of workplace exposures to chemical and biological agents. | |
Explanation | Copyright (c) 2022 Haz-Map(R). All rights reserved. Unless otherwise indicated, all materials from Haz-Map are copyrighted by Haz-Map(R). No part of these materials, either text or image may be used for any purpose other than for personal use. Therefore, reproduction, modification, storage in a retrieval system or retransmission, in any form or by any means, electronic, mechanical or otherwise, for reasons other than personal use, is strictly prohibited without prior written permission. | |
Color/Form |
Colorless monoclinic crystals, refractory solid | |
CAS No. |
12168-85-3 | |
Record name | Tricalcium silicate | |
Source | ChemIDplus | |
URL | https://pubchem.ncbi.nlm.nih.gov/substance/?source=chemidplus&sourceid=0012168853 | |
Description | ChemIDplus is a free, web search system that provides access to the structure and nomenclature authority files used for the identification of chemical substances cited in National Library of Medicine (NLM) databases, including the TOXNET system. | |
Record name | Calcium oxide silicate (Ca3O(SiO4)) | |
Source | EPA Chemicals under the TSCA | |
URL | https://www.epa.gov/chemicals-under-tsca | |
Description | EPA Chemicals under the Toxic Substances Control Act (TSCA) collection contains information on chemicals and their regulations under TSCA, including non-confidential content from the TSCA Chemical Substance Inventory and Chemical Data Reporting. | |
Record name | Tricalcium silicate | |
Source | EPA DSSTox | |
URL | https://comptox.epa.gov/dashboard/DTXSID6049804 | |
Description | DSSTox provides a high quality public chemistry resource for supporting improved predictive toxicology. | |
Record name | Tricalcium silicon pentaoxide | |
Source | European Chemicals Agency (ECHA) | |
URL | https://echa.europa.eu/substance-information/-/substanceinfo/100.032.112 | |
Description | The European Chemicals Agency (ECHA) is an agency of the European Union which is the driving force among regulatory authorities in implementing the EU's groundbreaking chemicals legislation for the benefit of human health and the environment as well as for innovation and competitiveness. | |
Explanation | Use of the information, documents and data from the ECHA website is subject to the terms and conditions of this Legal Notice, and subject to other binding limitations provided for under applicable law, the information, documents and data made available on the ECHA website may be reproduced, distributed and/or used, totally or in part, for non-commercial purposes provided that ECHA is acknowledged as the source: "Source: European Chemicals Agency, http://echa.europa.eu/". Such acknowledgement must be included in each copy of the material. ECHA permits and encourages organisations and individuals to create links to the ECHA website under the following cumulative conditions: Links can only be made to webpages that provide a link to the Legal Notice page. | |
Record name | Tricalcium silicate | |
Source | Hazardous Substances Data Bank (HSDB) | |
URL | https://pubchem.ncbi.nlm.nih.gov/source/hsdb/880 | |
Description | The Hazardous Substances Data Bank (HSDB) is a toxicology database that focuses on the toxicology of potentially hazardous chemicals. It provides information on human exposure, industrial hygiene, emergency handling procedures, environmental fate, regulatory requirements, nanomaterials, and related areas. The information in HSDB has been assessed by a Scientific Review Panel. | |
Melting Point |
2150 °C | |
Record name | Tricalcium silicate | |
Source | Hazardous Substances Data Bank (HSDB) | |
URL | https://pubchem.ncbi.nlm.nih.gov/source/hsdb/880 | |
Description | The Hazardous Substances Data Bank (HSDB) is a toxicology database that focuses on the toxicology of potentially hazardous chemicals. It provides information on human exposure, industrial hygiene, emergency handling procedures, environmental fate, regulatory requirements, nanomaterials, and related areas. The information in HSDB has been assessed by a Scientific Review Panel. | |
Disclaimer and Information on In-Vitro Research Products
Please be aware that all articles and product information presented on BenchChem are intended solely for informational purposes. The products available for purchase on BenchChem are specifically designed for in-vitro studies, which are conducted outside of living organisms. In-vitro studies, derived from the Latin term "in glass," involve experiments performed in controlled laboratory settings using cells or tissues. It is important to note that these products are not categorized as medicines or drugs, and they have not received approval from the FDA for the prevention, treatment, or cure of any medical condition, ailment, or disease. We must emphasize that any form of bodily introduction of these products into humans or animals is strictly prohibited by law. It is essential to adhere to these guidelines to ensure compliance with legal and ethical standards in research and experimentation.