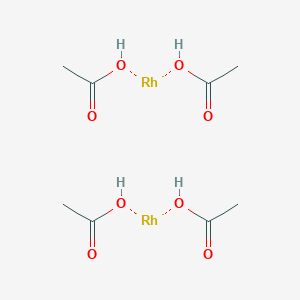
Dirhodium tetraacetate
Overview
Description
Dirhodium tetraacetate, [Rh₂(CH₃COO)₄], is a dinuclear Rh(II) complex with a paddlewheel structure. Its core consists of two Rh(II) ions bridged by four acetate ligands in the equatorial plane, leaving two axial coordination sites for ligands like water, amino acids, or other Lewis bases . This compound is notable for its unique Rh–Rh bond distance (2.3855 Å in [Rh₂(AcO)₄(H₂O)₂]), which is critical for its catalytic and biological activities . This compound exhibits antitumor properties by binding to DNA and inhibiting replication/transcription, as well as serving as a catalyst in organic synthesis for carbene transfer and cyclopropanation reactions .
Mechanism of Action
Target of Action
Dirhodium tetraacetate, also known as Rhodium(II) acetate dimer, primarily targets proteins and DNA. It has been found to extensively react with the model protein bovine pancreatic ribonuclease (RNase A) . The compound binds to the protein via coordination of the imidazole ring of a Histidine (His) side chain . Additionally, this compound has been shown to bind to B-DNA double helical structures .
Mode of Action
The interaction of this compound with its targets involves the coordination of the imidazole ring of a His side chain to one of its axial sites . This binding occurs while the dirhodium center and the acetato ligands remain unmodified . The compound extensively reacts with RNase A, indicating a strong interaction with this protein .
Biochemical Pathways
It’s known that the compound can break up the direct rh(ii)–rh(ii) bond and its carboxylate framework in the presence of glutathione, an antioxidant present in cells . This reaction could potentially affect various biochemical pathways, including those involving glutathione.
Result of Action
The binding of this compound to proteins and DNA can result in various molecular and cellular effects. For instance, the binding to RNase A could potentially affect the protein’s function . Additionally, the compound’s interaction with DNA could influence DNA structure and function . These interactions could potentially contribute to the compound’s cytotoxic activity .
Action Environment
The action, efficacy, and stability of this compound can be influenced by various environmental factors. For example, the presence of glutathione can lead to the breakdown of the compound’s Rh(II)–Rh(II) bond and carboxylate framework
Biochemical Analysis
Biochemical Properties
Dirhodium tetraacetate extensively reacts with proteins such as bovine pancreatic ribonuclease (RNase A) . The metal compound binds the protein via coordination of the imidazole ring of a His side chain to one of its axial sites, while the dirhodium center and the acetato ligands remain unmodified . This interaction provides valuable information for the design of artificial dirhodium-containing metalloenzymes .
Cellular Effects
This compound exhibits an appreciable carcinostatic activity, though lower than cisplatin .
Molecular Mechanism
The structure of the dirhodium/DNA adduct reveals a dimetallic center binding to an adenine via axial coordination . This interaction with DNA could play a crucial role in the antitumor properties of this compound .
Scientific Research Applications
Catalytic Applications
1.1 Catalysis in Organic Reactions
Dirhodium tetraacetate is recognized for its catalytic properties in several organic transformations. It serves as an effective catalyst for:
- Carbene Transfer Reactions : It facilitates the insertion of carbenes into C–H bonds and the formation of cyclopropanes from alkenes, which are crucial steps in organic synthesis .
- Hydrosilylation : The compound is employed in the hydrosilylation of alkynes, allowing for the formation of silanes from alkynes and silanes .
- Oxidation Reactions : It catalyzes the oxidation of alcohols to carbonyl compounds, showcasing its utility in synthetic organic chemistry .
Table 1: Summary of Catalytic Reactions Involving this compound
1.2 Metallaphotocatalysis
Recent studies have explored the use of this compound in metallaphotocatalysis, where it acts as a bifunctional catalyst. This involves merging carbenoid chemistry with photocatalytic processes to enhance reaction efficiency and selectivity . The compound's ability to absorb visible light makes it suitable for these applications, expanding its utility in synthetic methodologies.
Antitumor Activity
This compound has been investigated for its antitumor properties. Research indicates that it exhibits significant cytotoxic effects against various cancer cell lines, including leukemia and sarcoma models:
- Mechanism of Action : The compound appears to inhibit DNA synthesis in tumor cells and interacts with thiol groups in proteins, leading to oxidative modifications that disrupt cellular functions .
- In Vivo Studies : Animal studies have shown that treatment with this compound can increase survival times in tumor-bearing mice, suggesting its potential as a therapeutic agent .
Table 2: Summary of Antitumor Studies Involving this compound
Protein Interactions
This compound interacts with proteins, making it a valuable tool in biochemical research:
- Model Protein Studies : It has been used to study metalation processes with proteins like hen egg white lysozyme (HEWL), providing insights into how metal complexes can influence protein structure and function .
- Adduct Formation : The formation of stable adducts between this compound and proteins has been characterized, revealing structural details that inform the design of new biomaterials and therapeutic agents .
Table 3: Key Findings on Protein Interactions with this compound
Q & A
Q. What are the primary synthetic routes for dirhodium tetraacetate, and how is its purity validated?
This compound is synthesized via carboxylate-bridged dimerization of rhodium(II) acetate precursors. A common method involves refluxing rhodium(III) chloride with acetic acid and sodium acetate under inert conditions to form the paddlewheel structure . Purity is validated using:
- X-ray crystallography to confirm the Rh-Rh bond distance (~2.385 Å in the hydrated form) and ligand arrangement .
- UV-vis spectroscopy to detect axial ligand interactions (e.g., λmax shifts from 580 nm for water adducts to 650 nm for sulfur-containing ligands) .
- Elemental analysis to verify stoichiometry (C₈H₁₂O₈Rh₂) .
Q. How does this compound interact with sulfur-containing biomolecules, and what experimental methods are used to study these interactions?
This compound reacts with sulfur-containing amino acids (e.g., cysteine, methionine) via axial or equatorial ligand substitution, leading to structural decomposition or oligomeric Rh(III) species. Key methodologies include:
- ESI-MS : Monitors time-dependent species like [RhIII(SMC)₂]⁺ (SMC = S-methyl-L-cysteine) and dirhodium-methionine adducts .
- UV-vis kinetics : Tracks ligand substitution rates (e.g., methionine-induced Rh-Rh bond elongation from 2.385 Å to 2.54 Å) .
- X-ray crystallography : Resolves structural changes, such as Rh-S bond formation (2.255–2.266 Å) in methionine adducts .
Q. How does this compound bind to DNA, and how does this differ from cisplatin?
This compound binds B-DNA dodecamers via axial coordination to adenine bases, unlike cisplatin, which forms covalent crosslinks with guanine. Key distinctions:
- Crystallography : Dirhodium adducts retain the Rh-Rh bond (2.385 Å), while cisplatin disrupts DNA helicity .
- ESI-MS : Detects [Rh₂(OAc)₄-DNA] adducts (mass shift ~4086 Da) and monorhodium fragments after prolonged incubation .
- Thermodynamic stability : Dirhodium adducts exhibit slower dissociation rates compared to cisplatin’s labile aqua ligands .
Q. What catalytic applications does this compound have in organic synthesis?
This compound catalyzes enantioselective C-H insertions and cycloadditions :
- Diazo compound reactions : Mediates cyclopropanation and carbonyl ylide formation (e.g., ethyl diazoacetate + furan → bicyclic products) .
- Kinetic studies : Hammett analysis reveals a stepwise mechanism for O-H insertions (ρ = -0.5 to -1.2, indicating electrophilic transition states) .
- Ligand tuning : Chiral carboxylate ligands (e.g., (S)-MBA) enhance enantioselectivity (up to 95% ee) .
Q. How do temperature and ligand concentration affect this compound’s stability in biological systems?
- Temperature : At 40°C, methionine replaces acetate ligands, forming [Rh₂(OAc)₂(Met)₂]⁺ (m/z = 620.93). At 100°C, decomposition yields monomeric Rh(III) species (e.g., [RhIII(Met)₂]⁺, m/z = 398.99) .
- Ligand competition : Glutathione displaces methionine via stronger Rh-S bonding (ΔG ~ -25 kJ/mol), deactivating antitumor activity .
- pH dependence : At pH 4.8, S-methyl-L-cysteine induces Rh₂(OAc)₄ decomposition within 48 hours, forming [Rh(SMC)₃]⁺ .
Q. What computational tools are used to model this compound’s interactions with biomolecules?
- DFT calculations : Predict binding energies and orbital overlaps (e.g., glutathione’s thiolate-Rh σ-bonding destabilizes the Rh-Rh core) .
- Molecular mechanics : Simulates ligand substitution effects on Rh-Rh distances (e.g., sulfur ligands increase bond length by ~0.15 Å) .
- Docking studies : Identify preferential axial coordination sites on proteins (e.g., histidine residues in lysozyme) .
Q. How does this compound’s reactivity with proteins compare to diruthenium analogs?
- Bond stability : Dirhodium’s Rh-Rh bond breaks upon protein binding (e.g., lysozyme), whereas diruthenium retains its Ru-Ru bond .
- Coordination mode : Dirhodium prefers axial N/O donor sites, while diruthenium binds equatorial carboxylates .
- Structural studies : X-ray data show Rh₂(OAc)₄ forms 1:1 adducts with RNase A, unlike diruthenium’s polymeric networks .
Q. What safety precautions are critical when handling this compound?
- Toxicity : Classified as a target organ toxin (single/repeated exposure); use fume hoods and PPE .
- Disposal : Treat waste with 10% HNO₃ to precipitate rhodium, followed by filtration and landfill disposal .
- Ecological impact : Limited data on biodegradability; avoid aqueous release due to potential heavy metal accumulation .
Q. How is this compound’s antitumor activity modulated by cellular thiols?
- Thiol competition : Cysteine and glutathione degrade the paddlewheel structure via Rh-S bond formation, forming inactive Rh(III)-thiolate oligomers .
- Methionine protection : Weak axial methionine binding (Kd ~ 10⁻⁴ M) temporarily shields dirhodium from thiols, prolonging cytotoxicity .
- In vitro assays : MTT tests on L1210 cells show IC₅₀ increases 10-fold in glutathione-rich media .
Q. What advanced techniques resolve this compound’s structural dynamics in solution?
- Time-resolved XAFS : Tracks Rh-Rh bond elongation during ligand substitution (2.385 Å → 2.55 Å in methionine adducts) .
- Circular dichroism (CD) : Detects chiral ligand-induced Cotton effects (e.g., Δε = ±15 M⁻¹cm⁻¹ for (R)-MBA adducts) .
- Rigid-body refinement : Analyzes powder XRD data for coordination polymers (e.g., [Rh₂(OAc)₄·μ₂-Se₂C₅H₈]∞) .
Comparison with Similar Compounds
Comparison with Similar Dirhodium(II) Compounds
Structural and Bonding Differences
Catalytic Performance
- This compound excels in carbene transfer reactions (e.g., cyclopropanation) with turnover numbers (TON) exceeding 10⁴ .
- Dirhodium caprolactamate demonstrates exceptional regioselectivity in allylic oxidations, attributed to its electron-deficient core .
- Chiral dirhodium carboxamidates (e.g., Rh₂(Pro)₄) enable asymmetric catalysis but require bulky ligands to prevent racemization .
Key Research Findings
Ligand Exchange Dynamics
- Methionine displaces acetate ligands in this compound, forming [Rh₂(AcO)₂(Met)₂] with a Rh–Rh distance elongation to 2.55 Å. This is driven by the trans influence of sulfur coordination, which weakens the Rh–Rh bond via π-back donation .
- Cysteine induces structural breakdown, yielding insoluble Rh(III)-thiolate complexes, unlike methionine .
Spectroscopic Insights
- EXAFS data confirm axial sulfur coordination in Rh₂(AcO)₄–methionine adducts (Rh–S = 2.58 ± 0.02 Å), consistent with thioether-bonded Rh(II) complexes .
- ESI-MS reveals time-dependent ligand exchange: [Rh₂(AcO)₄(HMet)]⁺ (Day 1) → [Rh₂(AcO)₂(Met)₂]⁺ (Day 37) .
Protein Interactions
- This compound binds RNase A via His side chains, leaving the Rh₂ core intact .
- In contrast, lysozyme adducts show partial Rh₂ core fragmentation, highlighting protein-specific reactivity .
Data Tables
Table 1: Rh–Rh Bond Distances in Selected Complexes
Properties
CAS No. |
15956-28-2 |
---|---|
Molecular Formula |
C8H12O8Rh2 |
Molecular Weight |
441.99 g/mol |
IUPAC Name |
rhodium(2+);tetraacetate |
InChI |
InChI=1S/4C2H4O2.2Rh/c4*1-2(3)4;;/h4*1H3,(H,3,4);;/q;;;;2*+2/p-4 |
InChI Key |
SYBXSZMNKDOUCA-UHFFFAOYSA-J |
SMILES |
CC(=O)[O-].CC(=O)[O-].CC(=O)[O-].CC(=O)[O-].[Rh+2].[Rh+2] |
Canonical SMILES |
CC(=O)[O-].CC(=O)[O-].CC(=O)[O-].CC(=O)[O-].[Rh+2].[Rh+2] |
Key on ui other cas no. |
42204-14-8 15956-28-2 |
physical_description |
Green powder; Insoluble in water; [Alfa Aesar MSDS] |
Pictograms |
Irritant; Health Hazard |
Synonyms |
Tetrakis(μ-acetato)di-rhodium (Rh-Rh); Tetrakis[μ-(acetato-O:O’)]di-Rhodium(Rh-Rh); Bis(Rhodium diacetate); Dirhodium Tetraacetate; Dirhodium(II)tetraacetate; NSC 156310; Rhodium Acetate; Rhodium Diacetate Dimer; Rhodium(II) Acetate; Tetraacetatodir |
Origin of Product |
United States |
Synthesis routes and methods
Procedure details
Retrosynthesis Analysis
AI-Powered Synthesis Planning: Our tool employs the Template_relevance Pistachio, Template_relevance Bkms_metabolic, Template_relevance Pistachio_ringbreaker, Template_relevance Reaxys, Template_relevance Reaxys_biocatalysis model, leveraging a vast database of chemical reactions to predict feasible synthetic routes.
One-Step Synthesis Focus: Specifically designed for one-step synthesis, it provides concise and direct routes for your target compounds, streamlining the synthesis process.
Accurate Predictions: Utilizing the extensive PISTACHIO, BKMS_METABOLIC, PISTACHIO_RINGBREAKER, REAXYS, REAXYS_BIOCATALYSIS database, our tool offers high-accuracy predictions, reflecting the latest in chemical research and data.
Strategy Settings
Precursor scoring | Relevance Heuristic |
---|---|
Min. plausibility | 0.01 |
Model | Template_relevance |
Template Set | Pistachio/Bkms_metabolic/Pistachio_ringbreaker/Reaxys/Reaxys_biocatalysis |
Top-N result to add to graph | 6 |
Feasible Synthetic Routes
Disclaimer and Information on In-Vitro Research Products
Please be aware that all articles and product information presented on BenchChem are intended solely for informational purposes. The products available for purchase on BenchChem are specifically designed for in-vitro studies, which are conducted outside of living organisms. In-vitro studies, derived from the Latin term "in glass," involve experiments performed in controlled laboratory settings using cells or tissues. It is important to note that these products are not categorized as medicines or drugs, and they have not received approval from the FDA for the prevention, treatment, or cure of any medical condition, ailment, or disease. We must emphasize that any form of bodily introduction of these products into humans or animals is strictly prohibited by law. It is essential to adhere to these guidelines to ensure compliance with legal and ethical standards in research and experimentation.