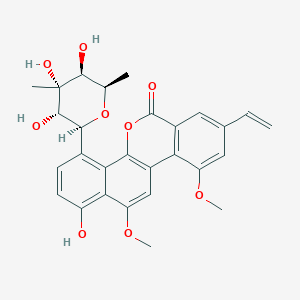
Chrysomycin A
Overview
Description
Chrysomycin A (CAS: 82196-88-1, MF: C28H28O9, MW: 508.5 g/mol) is a C-aryl glycoside antibiotic isolated from Streptomyces species . It exhibits dual biological activities:
- Antibacterial Activity: Inhibits DNA synthesis in bacteria, with a minimum inhibitory concentration (MIC) of 0.4 μg/mL against multi-drug-resistant Mycobacterium tuberculosis (MDR-TB) .
- Antitumor Activity: Suppresses tumor growth in murine models by targeting the Akt/GSK-3β/β-catenin signaling pathway, particularly in glioblastoma . Structurally, this compound features a tetracyclic aglycone core linked to a deoxy sugar moiety, a hallmark of flavinoid antibiotics . Its photoactivated DNA-crosslinking activity is hypothesized to involve interactions with histones and heat shock proteins .
Preparation Methods
Synthetic Routes and Reaction Conditions: Chrysomycin A can be synthesized through a 10-step scalable synthesis process. This process involves two sequential C-H functionalization steps and a late-stage C-glycosylation . The synthetic sequence has been optimized to facilitate the production of this compound and its analogues.
Industrial Production Methods: Industrial production of this compound involves the fermentation of Streptomyces species. Optimization of fermentation conditions, such as the concentration of glucose, corn starch, and soybean meal, can significantly enhance the yield . Mechanochemical preparation methods have also been developed to improve the solubility and oral bioavailability of this compound .
Chemical Reactions Analysis
Types of Reactions: Chrysomycin A undergoes various chemical reactions, including oxidation, reduction, and substitution. It is known to inhibit DNA synthesis in bacteria and suppress the growth of transplantable tumors in mice .
Common Reagents and Conditions: Common reagents used in the synthesis and modification of this compound include boronate esters, bromides, and aqueous sodium hydroxide . The reaction conditions often involve cross-coupling reactions and hydrolysis.
Major Products Formed: The major products formed from the reactions involving this compound include its derivatives, such as polycarcin V and gilvocarcin V, which have shown superior potency against MDR-TB .
Scientific Research Applications
Anticancer Properties
Mechanism of Action
Chr-A has been identified as a promising chemotherapeutic agent, particularly in the treatment of glioblastoma. It reshapes cellular metabolism and increases oxidative stress, which inhibits tumor growth. Studies have shown that Chr-A suppresses orthotopic glioblastoma (GBM) tumor growth in vivo and alters metabolic pathways associated with glucose and glutamate accumulation, thereby reducing tumor progression .
Cellular Effects
Research indicates that Chr-A effectively inhibits the proliferation, migration, and invasion of glioblastoma cells (U251 and U87-MG). It operates through the Akt/GSK-3β/β-Catenin signaling pathway, leading to decreased cell viability and increased cytotoxicity . Additionally, Chr-A has been shown to induce apoptosis in KRAS-mutant lung adenocarcinoma cells by targeting topoisomerase II (Topo II), a critical enzyme for DNA replication and repair .
Antimicrobial Activity
Bacterial Infections
Chr-A demonstrates potent antibacterial activity against Staphylococcus aureus, including multidrug-resistant strains. It acts as a multitarget agent, binding to enzymes involved in cell wall biosynthesis, such as GlmU and DapD, thereby disrupting bacterial growth and biofilm formation . This mechanism is particularly relevant given the increasing prevalence of antibiotic-resistant infections.
Efficacy Against Biofilms
The compound has shown remarkable efficacy in eradicating S. aureus biofilms both in vitro and in vivo. Its ability to disrupt established biofilms makes it a valuable candidate for treating persistent infections that are often resistant to conventional antibiotics .
Research Developments
Synthesis of Derivatives
Recent studies have focused on synthesizing derivatives of Chr-A to enhance its therapeutic properties. A scalable synthesis process has been developed, leading to compounds that exhibit improved activity against multi-drug-resistant tuberculosis (MDR-TB) . These advancements highlight the potential for developing new antibiotics based on the Chr-A scaffold.
Comprehensive Data Table
Mechanism of Action
Chrysomycin A exerts its effects by inhibiting DNA synthesis in bacteria and promoting DNA cross-linking with histone 3 and GRP78 when photoactivated by near-UV light . This mechanism is similar to that of gilvocarcin V, another C-aryl glycoside. The compound’s ability to inhibit topoisomerase II is crucial for its antibacterial and anticancer activities .
Comparison with Similar Compounds
Comparison with Structurally and Functionally Similar Compounds
Chrysomycin A belongs to the flavinoid antibiotic family, which includes Gilvocarcin V and Ravidomycin. These compounds share a tetracyclic aromatic backbone but differ in substituents and sugar moieties, leading to variations in bioactivity and mechanisms (Table 1) .
Structural Comparison
Key Structural Features:
Notable Differences:
- The C8 vinyl group in this compound enhances DNA intercalation compared to Gilvocarcin V’s methoxy group .
- Ravidomycin’s amino sugar (ravidosamine) improves solubility and cellular uptake, contributing to higher antitumor potency .
Functional and Mechanistic Comparison
Mechanistic Insights :
- This compound and Gilvocarcin V both require photoactivation to form DNA adducts, but this compound’s vinyl group enables stronger intercalation, enhancing DNA synthesis inhibition .
- Ravidomycin’s amino sugar moiety facilitates nuclear localization, increasing topoisomerase II inhibition efficacy compared to its deacetylated analogue .
Solubility and Stability
Biological Activity
Chrysomycin A (Chr-A) is a glycoside compound derived from Streptomyces species, first identified in 1955. It has gained attention for its diverse biological activities, particularly its anti-tumor, anti-tuberculosis, and anti-inflammatory properties. This article provides a comprehensive overview of the biological activity of this compound, focusing on its mechanisms of action, efficacy against various diseases, and notable research findings.
This compound exhibits its biological effects through several mechanisms:
- Inhibition of Cell Proliferation : Chr-A has been shown to significantly inhibit the proliferation of glioblastoma cells (U251 and U87-MG). It disrupts the cell cycle and promotes apoptosis via the Akt/GSK-3β signaling pathway. Research indicates that treatment with Chr-A leads to increased caspase 3/7 activity, which is critical for the apoptotic process .
- Induction of Apoptosis : Flow cytometry studies demonstrate that Chr-A increases the apoptotic ratio in glioblastoma cells. The compound alters the expression levels of apoptosis-related proteins, notably increasing Bax and decreasing Bcl-2, thus triggering a caspase cascade that leads to cell death .
- Anti-Migratory Effects : Chr-A not only inhibits cell proliferation but also reduces migration and invasion capabilities in glioblastoma cells. This is evidenced by Transwell assays showing a significant decrease in cell migration following treatment with varying concentrations of Chr-A .
- Antimicrobial Activity : In addition to its anti-cancer properties, Chr-A demonstrates potent antimicrobial effects, particularly against Mycobacterium tuberculosis. It has been reported to have a minimum inhibitory concentration (MIC) comparable to rifampicin, making it effective against both planktonic and intracellular strains of tuberculosis .
Efficacy Against Glioblastoma
Recent studies have highlighted the potential of this compound as a therapeutic agent against glioblastoma:
- In Vivo Studies : In xenograft models using hairless mice implanted with U87 glioma cells, Chr-A treatment resulted in significant tumor regression. The study identified key signaling pathways involved in its anti-tumor effects, including apoptosis and the PI3K-Akt pathway .
- Cell Viability Assays : EdU incorporation assays confirmed that Chr-A effectively inhibits DNA synthesis in glioblastoma cells, further supporting its role as a potent anti-cancer agent .
Comparative Efficacy
The following table summarizes the biological activities and efficacy of this compound against various pathogens and cancer cell lines:
Activity | Target Organism/Cell Line | Efficacy | Mechanism |
---|---|---|---|
Anti-Glioblastoma | U251, U87-MG | Significant inhibition of proliferation | Akt/GSK-3β signaling pathway |
Induction of Apoptosis | U251, U87-MG | Increased caspase 3/7 activity | Activation of mitochondrial apoptosis pathway |
Anti-Tuberculosis | Mycobacterium tuberculosis | MIC = 0.4 μg/mL | Disruption of bacterial cell function |
Antimicrobial | M. smegmatis, B. subtilis | Effective at low concentrations | Inhibition of cell wall synthesis |
Case Studies
- Glioblastoma Treatment : A study conducted on human glioma xenografts demonstrated that this compound not only inhibited tumor growth but also altered key apoptotic markers. The findings suggest that Chr-A could be a promising candidate for further development in glioblastoma therapy .
- Tuberculosis Management : Research has shown that this compound exhibits strong bactericidal activity against both drug-sensitive and multi-drug-resistant strains of Mycobacterium tuberculosis. This highlights its potential as an alternative treatment option in the face of rising antibiotic resistance .
Q & A
Basic Research Questions
Q. Q1. What are the primary mechanisms by which Chrysomycin A exerts its antitumor activity?
this compound inhibits cancer cell proliferation and migration via modulation of the Akt/GSK-3β/β-catenin signaling pathway , as demonstrated in glioblastoma models. To validate this mechanism:
- Perform Western blotting to assess phosphorylation levels of Akt and GSK-3β.
- Use β-catenin immunofluorescence to track nuclear translocation in treated vs. untreated cells.
- Conduct scratch/wound-healing assays to quantify migration inhibition .
Q. Q2. How can researchers optimize this compound yield during fermentation?
A Plackett-Burman Design (PBD) followed by Box-Behnken optimization is recommended:
- Screen critical media components (e.g., sodium chloride, calcium carbonate) using PBD with high (+1), low (-1), and intermediate (0) levels.
- Apply response surface methodology (RSM) to model interactions between factors (e.g., Y = β₀ + β₁X₁ + β₂X₂ + ...).
- Validate predictions via triplicate fermentation runs and HPLC quantification .
Q. Q3. What in vitro assays are most reliable for assessing this compound’s antibacterial activity?
- Minimum Inhibitory Concentration (MIC) assays against Gram-positive bacteria (e.g., Staphylococcus aureus).
- Time-kill kinetics to evaluate bactericidal vs. bacteriostatic effects.
- Pair with DNA synthesis inhibition assays (e.g., radiolabeled thymidine uptake) to confirm mechanism .
Advanced Research Questions
Q. Q4. How should researchers address contradictions in this compound’s reported efficacy across studies?
- Conduct meta-analysis of dose-response data, stratifying by cell type (e.g., A549 vs. glioblastoma) and treatment duration.
- Replicate experiments under standardized conditions (e.g., serum-free media, 48-hour exposure).
- Use sensitivity analysis to identify confounding variables (e.g., batch-to-batch compound variability) .
Q. Q5. What experimental designs are optimal for studying this compound’s synergy with other anticancer agents?
- Apply the Chou-Talalay combination index (CI) method :
- Treat cells with this compound and a partner drug (e.g., cisplatin) at fixed ratios.
- Calculate CI values using CompuSyn software; CI < 1 indicates synergy.
- Validate synergy via apoptosis assays (Annexin V/PI staining) and cell cycle profiling .
Q. Q6. How can researchers resolve inconsistencies in this compound’s DNA-binding behavior compared to structural analogs like Gilvocarcin V?
- Use circular dichroism (CD) spectroscopy to compare DNA conformational changes induced by both compounds.
- Perform molecular docking simulations (e.g., AutoDock Vina) to map binding sites on DNA minor/major grooves.
- Validate with topoisomerase II inhibition assays under near-UV light to assess photoactivation-dependent effects .
Q. Q7. What strategies mitigate challenges in reproducing this compound’s in vivo antitumor results?
- Standardize animal models (e.g., BALB/c nude mice with xenograft tumor volumes ≤ 100 mm³ at treatment initiation).
- Include positive controls (e.g., doxorubicin) and monitor pharmacokinetics (plasma half-life via LC-MS/MS).
- Address batch variability by sourcing this compound from ≥2 independent suppliers and verifying purity via NMR .
Q. Methodological Guidance
Q. Q8. How to formulate a robust research question for studying this compound’s novel applications?
Use the PICO framework :
- P opulation: Specific cancer cell line or bacterial strain.
- I ntervention: this compound dose/administration route.
- C omparison: Existing standard therapy (e.g., vancomycin for MRSA).
- O utcome: Quantifiable endpoints (e.g., 50% reduction in tumor volume).
Ensure alignment with FINER criteria (Feasible, Novel, Ethical, Relevant) .
Q. Q9. What statistical approaches are critical for analyzing this compound’s dose-response data?
- Fit data to logistic regression models (e.g., IC₅₀ calculation via GraphPad Prism).
- Apply ANOVA with Tukey’s post-hoc test for multi-group comparisons.
- Use principal component analysis (PCA) to identify latent variables in fermentation optimization studies .
Q. Data Reproducibility and Validation
Q. Q10. How to ensure reproducibility when scaling up this compound production?
- Document critical process parameters (CPPs) (e.g., pH, agitation speed) using a process analytical technology (PAT) framework.
- Perform design space exploration via RSM to define acceptable ranges for each factor.
- Validate with three consecutive batches meeting predefined quality thresholds (e.g., ≥95% purity) .
Q. Q11. What steps resolve discrepancies between in silico predictions and experimental results for this compound’s targets?
Properties
IUPAC Name |
8-ethenyl-1-hydroxy-10,12-dimethoxy-4-[(2S,3S,4R,5S,6R)-3,4,5-trihydroxy-4,6-dimethyloxan-2-yl]naphtho[1,2-c]isochromen-6-one | |
---|---|---|
Source | PubChem | |
URL | https://pubchem.ncbi.nlm.nih.gov | |
Description | Data deposited in or computed by PubChem | |
InChI |
InChI=1S/C28H28O9/c1-6-13-9-16-20(18(10-13)34-4)15-11-19(35-5)22-17(29)8-7-14(21(22)23(15)37-27(16)32)24-26(31)28(3,33)25(30)12(2)36-24/h6-12,24-26,29-31,33H,1H2,2-5H3/t12-,24+,25+,26+,28-/m1/s1 | |
Source | PubChem | |
URL | https://pubchem.ncbi.nlm.nih.gov | |
Description | Data deposited in or computed by PubChem | |
InChI Key |
OMDANBMKOUVKAG-WZNMFJNZSA-N | |
Source | PubChem | |
URL | https://pubchem.ncbi.nlm.nih.gov | |
Description | Data deposited in or computed by PubChem | |
Canonical SMILES |
CC1C(C(C(C(O1)C2=C3C(=C(C=C2)O)C(=CC4=C3OC(=O)C5=C4C(=CC(=C5)C=C)OC)OC)O)(C)O)O | |
Source | PubChem | |
URL | https://pubchem.ncbi.nlm.nih.gov | |
Description | Data deposited in or computed by PubChem | |
Isomeric SMILES |
C[C@@H]1[C@@H]([C@@]([C@H]([C@@H](O1)C2=C3C(=C(C=C2)O)C(=CC4=C3OC(=O)C5=C4C(=CC(=C5)C=C)OC)OC)O)(C)O)O | |
Source | PubChem | |
URL | https://pubchem.ncbi.nlm.nih.gov | |
Description | Data deposited in or computed by PubChem | |
Molecular Formula |
C28H28O9 | |
Source | PubChem | |
URL | https://pubchem.ncbi.nlm.nih.gov | |
Description | Data deposited in or computed by PubChem | |
Molecular Weight |
508.5 g/mol | |
Source | PubChem | |
URL | https://pubchem.ncbi.nlm.nih.gov | |
Description | Data deposited in or computed by PubChem | |
CAS No. |
82196-88-1 | |
Record name | Chrysomycin A | |
Source | ChemIDplus | |
URL | https://pubchem.ncbi.nlm.nih.gov/substance/?source=chemidplus&sourceid=0082196881 | |
Description | ChemIDplus is a free, web search system that provides access to the structure and nomenclature authority files used for the identification of chemical substances cited in National Library of Medicine (NLM) databases, including the TOXNET system. | |
Retrosynthesis Analysis
AI-Powered Synthesis Planning: Our tool employs the Template_relevance Pistachio, Template_relevance Bkms_metabolic, Template_relevance Pistachio_ringbreaker, Template_relevance Reaxys, Template_relevance Reaxys_biocatalysis model, leveraging a vast database of chemical reactions to predict feasible synthetic routes.
One-Step Synthesis Focus: Specifically designed for one-step synthesis, it provides concise and direct routes for your target compounds, streamlining the synthesis process.
Accurate Predictions: Utilizing the extensive PISTACHIO, BKMS_METABOLIC, PISTACHIO_RINGBREAKER, REAXYS, REAXYS_BIOCATALYSIS database, our tool offers high-accuracy predictions, reflecting the latest in chemical research and data.
Strategy Settings
Precursor scoring | Relevance Heuristic |
---|---|
Min. plausibility | 0.01 |
Model | Template_relevance |
Template Set | Pistachio/Bkms_metabolic/Pistachio_ringbreaker/Reaxys/Reaxys_biocatalysis |
Top-N result to add to graph | 6 |
Feasible Synthetic Routes
Disclaimer and Information on In-Vitro Research Products
Please be aware that all articles and product information presented on BenchChem are intended solely for informational purposes. The products available for purchase on BenchChem are specifically designed for in-vitro studies, which are conducted outside of living organisms. In-vitro studies, derived from the Latin term "in glass," involve experiments performed in controlled laboratory settings using cells or tissues. It is important to note that these products are not categorized as medicines or drugs, and they have not received approval from the FDA for the prevention, treatment, or cure of any medical condition, ailment, or disease. We must emphasize that any form of bodily introduction of these products into humans or animals is strictly prohibited by law. It is essential to adhere to these guidelines to ensure compliance with legal and ethical standards in research and experimentation.