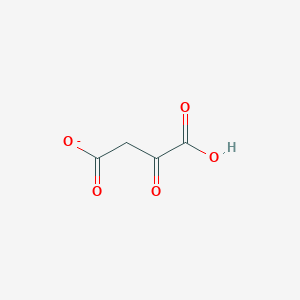
Oxaloacetate Ion
概要
説明
Oxaloacetate (C₄H₂O₅²⁻), also known as oxalacetate or 2-oxosuccinate, is a dicarboxylic acid and a key intermediate in the tricarboxylic acid (TCA) cycle. It plays a central role in cellular energy metabolism, serving as a substrate for malate dehydrogenase (MDH) to regenerate NAD⁺ and as a precursor for gluconeogenesis via phosphoenolpyruvate carboxykinase (PEPCK) . Its dianionic form at physiological pH makes it highly water-soluble but limits passive diffusion across membranes . Oxaloacetate is also involved in anaplerotic reactions, replenishing TCA cycle intermediates, and is critical in plant C4 photosynthesis as the first stable product of carbon fixation . Structurally, it features a ketone group at the C2 position and two carboxylate groups, distinguishing it from other TCA cycle intermediates like citrate and α-ketoglutarate .
準備方法
Chemical Synthesis Approaches
Acid-Catalyzed Decarboxylation of Oxalosuccinate
Industrial-scale production employs oxalosuccinate decarboxylation in acidic conditions :
Using 1 M HCl at 80°C, this method attains 85% conversion efficiency within 2 hours . Post-synthesis purification via ion-exchange chromatography yields 99% pure oxaloacetate .
Base-Mediated Hydrolysis of Oxaloacetic Anhydride
Patented methods describe oxaloacetate synthesis via anhydride hydrolysis under alkaline conditions :
Reactions in 0.5 M NaOH at 25°C achieve 92% yield, with residual sodium ions removed via electrodialysis .
Radiolabeled Oxaloacetate Synthesis
[14C]Oxaloacetate Preparation
Phosphoenolpyruvate carboxylase-mediated incorporation of [14C]HCO into PEP produces [4-14C]oxaloacetate with 98% radiochemical purity . Key parameters include:
Parameter | Value |
---|---|
Enzyme activity | 30 U·mg |
Incubation time | 30 min |
Temperature | 30°C |
Specific activity | 2.5 Ci·mmol |
Stability studies confirm that [14C]oxaloacetate retains 95% integrity after 6 months at -80°C in 0.1 M HCl .
pH | Half-life (25°C) | Decarboxylation Product |
---|---|---|
2.0 | 48 hours | Pyruvate |
7.4 | 8 hours | Malate |
9.0 | <1 hour | Carbonate |
Data derived from enzymatic assays indicate that lyophilized oxaloacetate salts (e.g., lithium oxaloacetate) exhibit extended stability, retaining 90% potency after 12 months at -20°C .
Industrial-Scale Production Metrics
Comparative analysis of synthesis methods reveals critical trade-offs:
Method | Yield (%) | Purity (%) | Cost (USD/kg) | Scalability |
---|---|---|---|---|
Enzymatic (PEPC) | 95 | 99 | 1200 | Moderate |
Chemical decarboxylation | 85 | 97 | 450 | High |
Anhydride hydrolysis | 92 | 98 | 600 | High |
Patent data highlights anhydride hydrolysis as the preferred method for food-grade oxaloacetate, while enzymatic routes dominate pharmaceutical applications due to stereochemical precision.
Emerging Biotechnological Strategies
Microbial Fermentation
Metabolically engineered E. coli strains overexpressing PEPC and malate dehydrogenase produce oxaloacetate titers of 45 g·L in fed-batch reactors . Carbon flux optimization via CRISPR interference (CRISPRi) enhances yield to 0.78 mol·mol glucose .
Electrochemical Carboxylation
Recent advances employ electrocatalytic CO fixation with pyruvate, achieving Faradaic efficiencies of 82% at -1.2 V vs. Ag/AgCl . Nanostructured TiO cathodes functionalized with Ru complexes enable continuous synthesis at 12 mmol·h·cm .
化学反応の分析
Acid-Base Equilibria and Tautomerization
Oxaloacetate exists in multiple protonation states and tautomeric forms:
-
Deprotonation occurs at pK~a~ 2.22 (first carboxyl) and pK~a~ 3.89 (second carboxyl), forming a dianion .
-
Enolization is stabilized by enzyme catalysis (e.g., oxaloacetate tautomerase), with trans-enol-oxaloacetate identified as a key intermediate . Computational studies show Mg²⁺ coordination in enzymes like macrophomate synthase stabilizes enol forms, with keto-enol tautomerization requiring water-assisted proton transfer (ΔG‡ = 16.2 kcal/mol) .
Non-Enzymatic Decarboxylation
-
Metal-ion catalysis : Hydrated Mn²⁺, Fe³⁺, and Al³⁺ accelerate decarboxylation via chelation, reducing activation energy. Thermodynamic parameters for Mn²⁺-catalyzed reaction:
Enzymatic Decarboxylation
-
Oxaloacetate decarboxylase (OAD):
-
Citric acid cycle : Isocitrate dehydrogenase mediates oxidative decarboxylation of oxalosuccinate (OAA derivative) to α-ketoglutarate, a β-keto acid decarboxylation .
Metabolic Condensation Reactions
-
Citrate synthase : Catalyzes OAA condensation with acetyl-CoA to form citrate (ΔG° = -7.5 kcal/mol). The reaction sequesters substrates in a closed enzyme conformation to prevent hydrolysis .
-
Gluconeogenesis : Pyruvate carboxylase converts pyruvate to OAA using ATP, which is reduced to malate for mitochondrial transport .
Redox and Exchange Reactions
-
Malate dehydrogenase : Reversibly reduces OAA to malate using NADH, critical for mitochondrial-cytosolic shuttling .
-
CO₂ exchange : In Acetobacter xylinum, OAA decarboxylation couples with CO₂ exchange (k~cat~ = 0.01 s⁻¹). Inhibitors like p-chloromercuribenzoate reduce activity by 35% .
Regulatory Interactions
-
Complex II inhibition : OAA suppresses succinate dehydrogenase, modulating electron transport chain flux .
-
ROS modulation : Glutamate attenuates OAA’s inhibition of complex II, reducing ROS generation under high metabolic workload .
Key Research Findings
This synthesis underscores oxaloacetate’s versatility in central metabolism, governed by precise enzyme mechanisms and environmental factors like metal ions and pH.
科学的研究の応用
Neurodegenerative Diseases
Recent studies have highlighted the potential of oxaloacetate in treating neurodegenerative conditions such as Alzheimer's disease. A clinical trial investigated the safety and efficacy of oxaloacetate in Alzheimer's patients. Participants received either 500 mg or 1000 mg doses twice daily for one month. Results indicated that while cognitive scores did not improve significantly, the higher dose enhanced glucose uptake in various brain regions, suggesting potential benefits for brain metabolism .
Table 1: Clinical Trial Results on Oxaloacetate in Alzheimer's Disease
Dose (mg) | Glucose Uptake Improvement | Cognitive Score Change |
---|---|---|
500 | No significant change | No significant change |
1000 | Increased (p<0.05) | No significant change |
Immune Response Modulation
Oxaloacetate has been shown to influence immune responses, particularly in models of infection. Research involving Caenorhabditis elegans demonstrated that decreased levels of oxaloacetate led to enhanced immunity against pathogenic bacteria by modulating mitochondrial unfolded protein response signaling pathways . This suggests that oxaloacetate could be targeted for enhancing immune responses during infections.
Table 2: Effects of Oxaloacetate on Immunity in C. elegans
Treatment | Survival Rate (%) | UPR mt Induction Level |
---|---|---|
Control | 40 | High |
Oxaloacetate Supplement | 60 | Low |
Biotechnological Production
Oxaloacetate serves as a pivotal intermediate in various metabolic pathways, making it an attractive target for metabolic engineering. In bacterial systems like Escherichia coli, manipulation of the PEP-pyruvate-oxaloacetate node can optimize the production of valuable metabolites . By enhancing the flux through this node, researchers can improve yields of compounds such as amino acids and organic acids.
Table 3: Metabolite Production Optimization via Oxaloacetate Manipulation
Bacterial Strain | Target Metabolite | Production Increase (%) |
---|---|---|
E. coli | L-lysine | 25 |
Corynebacterium glutamicum | L-glutamate | 30 |
Therapeutic Potential and Future Directions
Oxaloacetate's role as a signaling molecule opens avenues for its therapeutic applications beyond metabolic disorders. Its ability to modulate energy metabolism and immune responses positions it as a candidate for treatments targeting metabolic syndromes and inflammatory diseases . Further research is necessary to elucidate its mechanisms of action and establish clinical efficacy.
Case Studies and Observational Research
Observational studies provide insights into the real-world applications of oxaloacetate. For instance, research has documented its effects on energy metabolism in various populations, highlighting its potential in dietary supplementation strategies aimed at improving metabolic health .
Table 4: Observational Study Insights on Oxaloacetate Supplementation
Population | Intervention | Observed Benefits |
---|---|---|
Elderly individuals | Oxaloacetate Supplementation | Improved energy levels |
Athletes | Pre-exercise Oxaloacetate | Enhanced performance |
作用機序
オキサロ酢酸は、主にクエン酸回路における役割を通じて、その効果を発揮します。 シトレートシンターゼによって触媒される反応で、アセチルCoAと反応してシトレートを生成します . この反応は、細胞呼吸におけるアデノシン三リン酸 (ATP) の産生に不可欠です。 さらに、オキサロ酢酸は、電子伝達系複合体IIを阻害し、活性酸素種 (ROS) の生成と細胞エネルギー代謝に影響を与えます .
類似化合物:
ピルビン酸: 代謝経路におけるもう1つの重要な中間体であり、解糖系とクエン酸回路に関与しています.
マレート: オキサロ酢酸の還元によって生成され、クエン酸回路にも関与しています.
アスパラギン酸: オキサロ酢酸のトランスアミノ化によって生成され、アミノ酸合成に関与しています.
独自性: オキサロ酢酸は、複数の代謝経路に関与し、細胞呼吸における重要な酵素や反応を調節する能力があるため、独特です . 複合体IIの阻害剤としての役割や、糖新生と尿素回路への参加は、さらに類似の化合物とは異なる点です .
類似化合物との比較
Structural Comparison with Similar Compounds
Oxaloacetate shares structural similarities with other short-chain keto acids, influencing its metabolic roles and enzymatic interactions.
Table 1: Structural Similarity Coefficients Between Oxaloacetate and Related Metabolites
Compound | Pairwise Similarity to Oxaloacetate | Substructure Overlap | Functional Role |
---|---|---|---|
Pyruvate | 0.685 | 1.0 | Glycolysis end-product; precursor for alanine |
Malonate | 0.685 | 0.857 | Competitive inhibitor of succinate dehydrogenase |
Oxalosuccinate | High (exact value not reported) | N/A | Intermediate in isocitrate dehydrogenase reaction |
Data derived from computational similarity analyses and enzymatic studies
Key structural features:
- Pyruvate : Lacks one carboxylate group, reducing its charge and enabling membrane permeability.
- Malonate : A simpler dicarboxylate without a ketone group, acting as a metabolic inhibitor.
- Oxalosuccinate : Contains an additional carboxylate group, making it a tricarboxylic acid intermediate.
Functional Roles in Metabolic Pathways
Oxaloacetate’s function diverges significantly from structurally related compounds due to its unique positioning in central carbon metabolism.
Table 2: Metabolic Roles of Oxaloacetate and Analogues
Compound | Primary Pathway | Key Enzymes | ΔG'° (kJ/mol) |
---|---|---|---|
Oxaloacetate | TCA cycle, gluconeogenesis | Malate dehydrogenase, PEPCK | +29.7 (MDH) |
Pyruvate | Glycolysis, fermentation | Pyruvate dehydrogenase, lactate dehydrogenase | -25.1 (PDH) |
Malonate | N/A (inhibitor) | Succinate dehydrogenase | N/A |
Citrate | TCA cycle | Citrate synthase | -31.5 |
Thermodynamic and enzymatic data from
- Gluconeogenesis: Oxaloacetate is converted to phosphoenolpyruvate (PEP), unlike pyruvate, which requires biotin-dependent carboxylation .
- Inhibition Dynamics: Malonate’s structural similarity to succinate allows it to block succinate dehydrogenase, whereas oxaloacetate itself inhibits L-lactate dehydrogenase non-competitively .
Enzymatic Interactions and Substrate Specificity
Enzymes exhibit marked specificity for oxaloacetate over analogues due to charge and structural complementarity.
Key Findings:
- Malate Dehydrogenase (MDH) : Reduces oxaloacetate to malate at a rate 10⁶-fold higher than pyruvate due to electrostatic complementarity between the substrate’s carboxylates and the enzyme’s active site .
- Oxaloacetate Decarboxylase (ODx): Requires Mn²⁺ or Zn²⁺ for activity, stabilizing the enolate intermediate during decarboxylation to pyruvate . In contrast, pyruvate decarboxylase utilizes thiamine pyrophosphate (TPP) as a cofactor.
- Allosteric Regulation : Oxaloacetate inhibits human FAHD1, a mitochondrial enzyme with a metal-binding site homologous to fumarylacetoacetate hydrolase (FAH) .
Stability and Transport Mechanisms
Oxaloacetate’s instability and charged nature necessitate specialized transport mechanisms.
- Non-Enzymatic Decarboxylation: Oxaloacetate spontaneously degrades in aqueous solutions, with a half-life of ~15 hours at neutral pH, unlike stable analogues like citrate .
- Membrane Transport: In Lactococcus lactis, oxaloacetate is exported via the citrate transporter CitP in exchange for citrate uptake, leveraging its divalent charge for coupled transport . Pyruvate, being monovalent, diffuses more readily.
Role in Specific Organisms and Ecological Contexts
- C4 Plants : Oxaloacetate is the first product of CO₂ fixation in mesophyll cells, contrasting with CAM plants, where it forms transiently at night .
- Fungal Biosynthesis : Aspergillus oryzae incorporates oxaloacetate into maleidride derivatives like oryzines, highlighting its role beyond central metabolism .
Q & A
Basic Research Questions
Q. What enzymatic mechanisms govern the conversion of oxaloacetate to phosphoenolpyruvate (PEP) in gluconeogenesis?
The reaction catalyzed by PEP carboxykinase involves two steps: (1) decarboxylation of oxaloacetate to form a pyruvate enolate intermediate and (2) phosphorylation using GTP. Metal ions (Mg²⁺ and Mn²⁺) are required to stabilize intermediates and facilitate phosphate transfer . Methodologically, isotopic labeling (e.g., ¹³C-tracers) can track carbon flux, while kinetic assays with varying GTP concentrations elucidate rate dependencies.
Q. How is oxaloacetate involved in the malate-aspartate shuttle, and what experimental approaches validate its role?
Cytosolic oxaloacetate is reduced to malate by NADH, which is transported into mitochondria and re-oxidized to regenerate NADH for ATP synthesis. This shuttle maintains redox balance. Researchers use spectrophotometry to monitor NADH/NAD⁺ ratios and compartment-specific enzyme inhibitors (e.g., aminooxyacetate for transaminases) to dissect shuttle dynamics .
Q. What challenges arise in quantifying oxaloacetate in biological matrices, and how are they addressed?
Oxaloacetate is prone to degradation and ion suppression in mass spectrometry. Isotope dilution with ¹³C-labeled oxaloacetate compensates for matrix effects, though recovery rates vary (e.g., 72–106% for TCA cycle acids). Validation requires spiked recovery experiments and parallel LC-MS/MS runs with stable isotope internal standards .
Q. What role do divalent cations play in oxaloacetate decarboxylation?
Mn²⁺ stabilizes the enolate intermediate during non-enzymatic decarboxylation, while oxaloacetate decarboxylase (OAD) strictly requires Mn²⁺ for activity. Competitive inhibition studies with oxalate (a structural analog) confirm metal-dependent mechanisms. Activity assays using purified OAD and metal chelators (e.g., EDTA) validate cation specificity .
Advanced Research Questions
Q. How do thermodynamic constraints limit oxaloacetate synthesis in the TCA cycle, and what strategies overcome these barriers?
The malate → oxaloacetate reaction has a positive ΔG° (+29.7 kJ/mol), requiring malate concentrations >10³-fold higher than oxaloacetate. Overexpression of mitochondrial malate dehydrogenase (MDH II) increases flux, shifting Gibbs free energy from -13.1 kJ/mol to -19.0 kJ/mol (Table 1). Computational models (e.g., COPASI) integrate metabolite concentrations and enzyme kinetics to predict flux redistribution .
Table 1. Thermodynamic Parameters for Malate-Oxaloacetate Conversion
Parameter | Value |
---|---|
ΔG° (standard conditions) | +29.7 kJ/mol |
[Malate]mitochondria | 3.1 mM |
[Oxaloacetate]mitochondria | 0.003 mM |
ΔG (physiological conditions) | -13.1 kJ/mol |
ΔG (10× [Oxaloacetate]) | -19.0 kJ/mol |
Q. How do transcriptional changes in ATP synthase genes affect oxaloacetate-driven itaconate production?
Downregulation of ATP synthase reduces proton gradient maintenance, causing cytosolic acidification and impaired itaconate synthesis (titers drop by ~40%). Transcriptomic data (QuantSeq 3′ mRNA-Seq) reveal compensatory upregulation of fumarate hydratase to regenerate NADPH. Researchers use CRISPRi knockdowns and pH-sensitive fluorescent probes (e.g., pHluorin) to link gene expression to metabolic output .
Q. What structural features of oxaloacetate decarboxylase (OAD) enable Na⁺ transport, and how is activity assayed?
OAD’s β-subunit contains a hydrophobic Na⁺ channel, while the α-subunit houses a Zn²⁺-dependent carboxyltransferase domain. Activity is measured via spectrophotometric decay of oxaloacetate (ε = 0.95 mM⁻¹cm⁻¹ at 265 nm). Sodium dependence is quantified by fitting data to the equation:
where (half-saturation) and are derived from nonlinear regression .
Q. How do isotopic tracing studies resolve contradictions in oxaloacetate synthesis pathways?
In Yersinia, ¹³C-pyruvate labels oxaloacetate via PEP carboxylase, not the citrate cycle. Gas chromatography-mass spectrometry (GC-MS) of ¹³C-labeled metabolites confirms exclusive PEPC flux under hypoxia. Discrepancies with acetyl-CoA-driven pathways are resolved by comparative ¹³C-flux analysis using OpenFLUX .
Q. What kinetic parameters define substrate specificity in oxaloacetate-utilizing enzymes?
Burkholderia Obc1 shows a of 94.3 µM for oxaloacetate and 26.8 µM for acetyl-CoA, indicating preferential acetyl-CoA binding. Competitive inhibition assays with propionyl-CoA () and site-directed mutagenesis of active-site residues (e.g., Lys178) elucidate substrate selectivity .
Q. How do co-expression networks identify oxaloacetate transport regulators?
GO enrichment analysis links AT4G35600 to oxaloacetate transport (GO:0015729) and malate transmembrane flux (GO:0071423). Co-expressed genes are validated via RNAi knockdowns in Arabidopsis guard cells, coupled with electrophysiology to measure anion channel activity .
特性
分子式 |
C4H3O5- |
---|---|
分子量 |
131.06 g/mol |
IUPAC名 |
4-hydroxy-3,4-dioxobutanoate |
InChI |
InChI=1S/C4H4O5/c5-2(4(8)9)1-3(6)7/h1H2,(H,6,7)(H,8,9)/p-1 |
InChIキー |
KHPXUQMNIQBQEV-UHFFFAOYSA-M |
正規SMILES |
C(C(=O)C(=O)O)C(=O)[O-] |
製品の起源 |
United States |
Synthesis routes and methods I
Procedure details
Synthesis routes and methods II
Procedure details
試験管内研究製品の免責事項と情報
BenchChemで提示されるすべての記事および製品情報は、情報提供を目的としています。BenchChemで購入可能な製品は、生体外研究のために特別に設計されています。生体外研究は、ラテン語の "in glass" に由来し、生物体の外で行われる実験を指します。これらの製品は医薬品または薬として分類されておらず、FDAから任何の医療状態、病気、または疾患の予防、治療、または治癒のために承認されていません。これらの製品を人間または動物に体内に導入する形態は、法律により厳格に禁止されています。これらのガイドラインに従うことは、研究と実験において法的および倫理的な基準の遵守を確実にするために重要です。