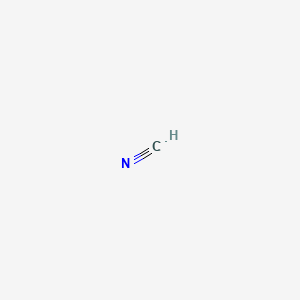
Hydrogen cyanide
概要
説明
Hydrogen cyanide (HCN), also known as prussic acid, is a colorless, highly volatile liquid or gas with a faint bitter almond odor. Its chemical formula is HCN, with a molecular weight of 27.0253 g/mol and a boiling point of 25.6–26°C, slightly above room temperature . HCN is weakly acidic (pKa = 9.2) and dissolves readily in water to form hydrocyanic acid .
科学的研究の応用
Industrial Applications
1. Chemical Manufacturing
Hydrogen cyanide serves as a precursor for several important chemicals:
- Sodium Cyanide and Potassium Cyanide : These compounds are primarily used in gold and silver mining for ore extraction through cyanidation processes. Sodium cyanide is also utilized in electroplating to enhance the durability and conductivity of metals .
- Adiponitrile : HCN is used in the production of adiponitrile, which is a key intermediate in the synthesis of Nylon-6,6. The hydrocyanation of butadiene with this compound leads to this compound, which is essential for producing various synthetic fibers and plastics .
- Acrylates and Methacrylates : HCN is involved in the production of acrylic plastics through acetone cyanohydrin, which is derived from HCN. This application accounts for a significant portion of HCN usage .
2. Fumigation
This compound is employed as a fumigant in agricultural settings and food production facilities. It effectively controls pest insects while minimizing environmental impact compared to other fumigants like sulfuryl fluoride and methyl bromide . The application methods typically involve sealed environments to ensure effective pest control.
Environmental Applications
1. Sensing and Detection
Recent advancements have led to the development of sensors that detect this compound vapors in real-time. For instance, portable ion mobility spectrometers can quantify HCN concentrations quickly, making them valuable tools for monitoring environmental pollutants . This capability is crucial for ensuring safety in industrial settings where HCN exposure may occur.
2. Bioremediation
Research indicates that certain bacteria can produce this compound as part of their metabolic processes, potentially aiding in the transformation of mercury compounds in contaminated environments. These bacteria may use HCN to inhibit non-producing competitors, thereby playing a role in bioremediation strategies .
Case Studies
Q & A
Basic Research Questions
Q. How is hydrogen cyanide used as a tracer for dense molecular gas in star-forming regions?
HCN’s rotational transitions (e.g., J=1→0, J=3→2) are sensitive to high-density environments (~10⁴–10⁶ cm⁻³), making it ideal for probing dense molecular clouds. Its hyperfine structure, caused by nitrogen’s nuclear quadrupole moment, allows estimation of optical depth and excitation temperatures via relative line intensities under local thermodynamic equilibrium (LTE) assumptions. Deviations from LTE ratios (e.g., 1:5:3 for J=3→2) indicate non-equilibrium conditions, such as radiative trapping or collisional effects .
Q. What experimental approaches determine the optical depth of HCN in interstellar clouds?
Optical depth is derived by comparing observed hyperfine line ratios to LTE predictions. For example, in HCN J=1→0, the satellite-to-main line ratio (R02 = I(ΔF=1)/I(ΔF=0)) is analyzed. Ratios lower than theoretical values (e.g., 1:25:1 for unresolved lines) suggest high optical depth, while anomalies may require non-LTE radiative transfer models (e.g., RADEX) to account for line overlap or velocity gradients .
Q. What are standard field and laboratory methods for detecting HCN in environmental samples?
Field detection uses portable meters or colorimetric kits to measure free cyanide ions (CN⁻). Laboratory analysis via gas chromatography-mass spectrometry (GC-MS) or infrared spectroscopy quantifies total cyanide, including bound forms. The EPA’s Environmental Response Laboratory Network (ERLN) standardizes methods for complex matrices (e.g., soil, water), requiring site-specific sampling plans to address reactivity and volatility .
Q. How do researchers account for HCN’s hyperfine structure in column density calculations?
Hyperfine splitting is modeled using radiative transfer codes (e.g., LAMDA database) that integrate collisional rates, Einstein coefficients, and excitation conditions. Column densities are derived from integrated line intensities, corrected for beam dilution and optical depth effects. Anomalous line ratios (e.g., enhanced ΔF=0 components) may necessitate non-LTE treatments .
Q. What thermodynamic data are critical for modeling HCN’s behavior in astrochemical simulations?
Key parameters include standard formation enthalpy (ΔfH° = 135.1 kJ/mol), entropy (S° = 201.8 J/mol·K), and reaction thermochemistry (e.g., HCN + OH → CN + H₂O). NIST’s curated datasets provide phase-specific properties (gas, condensed) and ion energetics, essential for kinetic models of interstellar or planetary atmospheres .
Advanced Research Questions
Q. What methodologies analyze hyperfine line anomalies in HCN transitions within star-forming cores?
Observational studies (e.g., TRAO, JCMT) use Gaussian decomposition of blended hyperfine lines (e.g., J=1→0 in low-mass cores) to calculate intensity ratios (R02, R12). For high-mass cores with turbulent broadening, velocity-resolved fitting identifies line overlap effects. Statistical tools like χ² minimization compare observed ratios to LTE predictions, revealing anomalies linked to radiative transfer effects or kinematics .
Q. How do HCN hyperfine line ratios vary between low-mass and high-mass star-forming cores?
Low-mass cores (e.g., Taurus-Auriga) show suppressed satellite lines (R02 < 0.2) due to high optical depth and subthermal excitation. High-mass cores (e.g., G333) exhibit near-LTE ratios (1:5:3 for J=3→2) due to turbulence-driven line overlap. These differences inform dynamical models: low-mass cores favor static collapse, while high-mass cores suggest supersonic infall .
Q. How can spectroscopic detection of HCN in exoplanetary atmospheres constrain carbon-to-oxygen (C/O) ratios?
Hubble/WFC3 observations of 55 Cancri e detected HCN absorption at 1.4–1.6 μm, indicating a C/O ratio >1. Atmospheric models (e.g., VULCAN) simulate photochemical pathways, where HCN abundance peaks in carbon-rich, oxygen-poor environments. Future JWST/NIRSpec observations will validate these models by resolving HCN’s rotational-vibrational bands .
Q. What mechanisms explain discrepancies between HCN hyperfine ratios and thermal equilibrium predictions?
In prestellar cores, line overlap in higher-J transitions (e.g., J=3→2) causes radiative pumping, redistributing population among hyperfine states. Non-LTE models incorporating collisional rate uncertainties (e.g., HCN-H₂ collisions) and velocity gradients reconcile anomalies, showing that ΔF=0 components are enhanced in infalling regions .
Q. How do advanced radiative transfer models reconcile HCN J=3→2 anomalies with core collapse velocities?
Non-LTE codes (e.g., RADEX) simulate line profiles using density, temperature, and velocity gradients. For example, in L1544, asymmetric hyperfine line profiles match models with infall speeds of 0.1 km/s, while high-mass cores require additional turbulence (Δv > 2 km/s) to explain blended components .
Q. What role does HCN play in tracing nitrogen chemistry in protoplanetary disks?
ALMA observations of disks (e.g., TW Hydrae) detect HCN isotopologues (H¹³CN, HC¹⁵N), revealing N/H ratios via isotopic fractionation. Chemical models show HCN forms via gas-phase reactions (N + CH₂ → HCN + H) or ice-phase hydrogenation of CN, with disk temperature gradients regulating pathways .
Q. Data Contradiction Analysis
Q. How can conflicting interpretations of HCN hyperfine ratios in prestellar cores be resolved?
Studies report weak correlations between HCN anomalies and core density (e.g., Crapsi et al. 2005). Contradictions arise from differing beam sizes (misaligned density tracers) or unaccounted velocity fields. Resolving these requires interferometric maps (e.g., ALMA) to isolate core kinematics and compare HCN ratios with independent tracers (e.g., N₂H⁺) .
Q. Why do some high-mass cores exhibit LTE-like HCN ratios despite high turbulence?
Turbulence broadens hyperfine lines, reducing optical depth and masking anomalies. However, in G333, high-resolution spectra reveal residual non-LTE effects (e.g., R02 = 0.3 vs. LTE 0.2), attributed to small-scale velocity substructure. Combining HCN with optically thin tracers (e.g., C¹⁸O) isolates density-driven effects .
Q. Methodological Resources
類似化合物との比較
Key Properties:
Property | Value | Source |
---|---|---|
Molecular Weight | 27.0253 g/mol | |
Boiling Point | 25.6–26°C | |
Density | 0.6876 g/cm³ | |
Melting Point | -13.4°C | |
Henry’s Law Constant | 6.9 × 10⁻⁵ atm·m³/mol (25°C) |
HCN is acutely toxic, with an LC50 (inhaled) of 100–300 ppm for humans . It inhibits cellular respiration by binding to cytochrome c oxidase, causing rapid systemic hypoxia . Industrially, HCN is used in electroplating, chemical synthesis (e.g., acrylonitrile), and as a ligand in metal coordination chemistry .
Hydrogen Cyanide vs. Hydrogen Sulfide (H₂S)
Both HCN and H₂S are volatile toxic gases, but their mechanisms of toxicity differ:
- Toxicity :
- Biological Effects :
- Physical Properties :
Property | HCN | H₂S |
---|---|---|
Boiling Point | 25.6°C | -60°C |
Molecular Weight | 27.03 g/mol | 34.08 g/mol |
Odor | Bitter almond | Rotten eggs |
This compound vs. Acetaldehyde (CH₃CHO)
HCN and acetaldehyde share inhibitory effects on microbial growth but differ chemically:
- Mechanism :
- Applications :
- Regulatory Limits :
Comparison with Other Cyanide Compounds
Cyanogen (C₂N₂):
- Structure : A dimer of CN radicals (N≡C–C≡N).
- Toxicity : Less acutely toxic than HCN but decomposes into HCN upon hydrolysis .
Cyanogen Chloride (ClCN):
- Reactivity : Highly reactive; used in chemical warfare.
- Toxicity : Causes lacrimation and pulmonary edema, unlike HCN’s rapid systemic effects .
Thiocyanate (SCN⁻):
- Detoxification Role : Formed during HCN metabolism via the enzyme rhodanese.
- Lower Toxicity : LD₅₀ (oral, rat) = 764 mg/kg vs. HCN’s 3.7 mg/kg .
準備方法
Industrial-Scale Synthesis Methods
The Andrussow Oxidation Process
The Andrussow process, developed by Leonid Andrussow in the 1920s, remains the dominant industrial method for HCN production. It involves the catalytic oxidation of methane (CH₄) and ammonia (NH₃) in the presence of oxygen (O₂) at temperatures exceeding 1,200°C . The reaction proceeds as:
4 + 2\text{NH}3 + 3\text{O}2 \rightarrow 2\text{HCN} + 6\text{H}2\text{O}
A platinum-rhodium alloy catalyst (typically 90% Pt, 10% Rh) is employed in the form of wire gauze with a surface area of 1,024 meshes/cm² . Reactors are designed as vertical tubes (2 m length, 16–18 mm diameter) to optimize gas residence time and heat transfer . The process achieves a yield of 85–90% relative to methane input, with annual outputs ranging from 5,000 to 20,000 metric tons per reactor . Energy consumption is approximately 40 MJ per kilogram of HCN, primarily due to the endothermic nature of ammonia dissociation .
Key Challenges :
-
High operating temperatures necessitate costly reactor materials.
-
Catalyst deactivation occurs every 10,000–15,000 hours, requiring replacement .
-
CO₂ emissions from methane combustion remain an environmental concern .
The BMA (Degussa) Process
The BMA method, commercialized by Degussa, eliminates oxygen but requires indirect heating via reactor walls . The reaction is:
4 + \text{NH}3 \rightarrow \text{HCN} + 3\text{H}_2 \quad (\Delta H = +251 \, \text{kJ/mol})
Operating at 1,200–1,300°C, this endothermic process uses nickel-based catalysts and achieves comparable yields to the Andrussow method (85–90%) . A notable advancement is the use of monolithic countercurrent reactors, which halve energy consumption to ~20 MJ/kg HCN . However, scalability is limited by heat transfer inefficiencies and higher capital costs.
The Shawinigan Process
This lesser-used method employs propane (C₃H₈) instead of methane, reacting with ammonia at 1,500°C over silica or alumina catalysts :
3\text{H}8 + \text{NH}3 \rightarrow 3\text{HCN} + 7\text{H}2
While offering higher HCN yields per hydrocarbon molecule, the Shawinigan process is economically viable only in regions with abundant propane supply.
Table 1: Comparative Analysis of Industrial HCN Synthesis Methods
Parameter | Andrussow Process | BMA Process | Shawinigan Process |
---|---|---|---|
Temperature (°C) | 1,200 | 1,200–1,300 | 1,500 |
Catalyst | Pt-Rh gauze | Nickel alloys | Silica/Alumina |
Yield (% CH₄ basis) | 85–90 | 85–90 | 70–75 |
Energy (MJ/kg HCN) | 40 | 20 | 45 |
Scale (tons/yr) | 5,000–20,000 | 1,000–5,000 | <1,000 |
Laboratory-Scale and Alternative Methods
Acidification of Cyanide Salts
Small quantities of HCN are generated by treating alkali cyanides (e.g., KCN) with strong acids :
The NIOSH Method 6010 standardizes this approach using 0.1 N NaOH for cyanide dissolution and barbituric acid-pyridine reagents for spectrophotometric detection . While simple, this method poses significant safety risks due to HCN volatility.
Corona Discharge Synthesis
A patent-pending method (US6096173A) enables non-catalytic HCN production at 500–1,000°C using a corona discharge . Methane and ammonia (molar ratio 1:1.001–1.1) are exposed to electric fields (10–20 kV), generating reactive radicals:
4 + \text{NH}3 \xrightarrow{\text{Corona}} \text{HCN} + 3\text{H}_2
This approach reduces energy consumption to 15 MJ/kg HCN and is suitable for medium-scale production (up to 1,000 tons/year) .
Emerging Innovations: Plasma-Assisted Synthesis
Recent advancements in dielectric barrier discharge (DBD) plasma reactors enable direct HCN synthesis from methane and nitrogen at <300°C :
4 + \text{N}2 \xrightarrow{\text{Plasma}} \text{HCN} + \text{H}2 + \text{C}2\text{H}_6
Key advantages include:
-
72% HCN yield at atmospheric pressure.
-
Elimination of ammonia, reducing upstream emissions.
-
Modular reactors for decentralized production.
Microkinetic models attribute selectivity to methyl radical (·CH₃) intermediates, which combine with nitrogen species to form HCN .
Historical Context and Evolution
George Beilby’s 1892 method—passing ammonia over glowing coal—laid the groundwork for modern HCN synthesis . Hamilton Castner’s 1894 sodium cyanide process (NaCN + H₂SO₄ → HCN + NaHSO₄) dominated early 20th-century production until catalytic gas-phase methods emerged .
Environmental and Economic Considerations
The Andrussow process accounts for 75% of global HCN capacity but emits 2.5 kg CO₂ per kg HCN . Plasma-assisted methods promise near-zero emissions but require scaling. Current production costs range from $1.20–$1.50/kg for industrial methods versus $5–$10/kg for laboratory-scale acidification .
特性
CAS番号 |
74-90-8 |
---|---|
分子式 |
HCN CHN |
分子量 |
27.025 g/mol |
IUPAC名 |
formonitrile |
InChI |
InChI=1S/CHN/c1-2/h1H |
InChIキー |
LELOWRISYMNNSU-UHFFFAOYSA-N |
SMILES |
C#N |
正規SMILES |
C#N |
沸点 |
78.1 °F at 760 mm Hg (EPA, 1998) 78 °F at 760 mm Hg (96%) (NIOSH, 2016) 25.6 °C 25.63 °C 26 °C 78°F (96%) |
Color/Form |
Colorless gas or liquid Water-white liquid below 26.5 °C Colorless or pale-blue liquid or gas (above 78 °F) ... [Note: Often used as a 96% solution in water] |
密度 |
0.699 (EPA, 1998) 0.69 (NIOSH, 2016) 0.6875 g/cu cm at 20 °C Relative density (water = 1): 0.69 (liquid) 0.69 |
引火点 |
0 °F (EPA, 1998) 0 °F (96%) (NIOSH, 2016) 0 °F (-18 °C) (Closed cup) -18 °C c.c. 0°F (96%) |
melting_point |
7.9 °F (EPA, 1998) 7 °F (96%) (NIOSH, 2016) -13.4 °C -13.28 °C -13 °C 7°F (96%) |
Key on ui other cas no. |
74-90-8 |
物理的記述 |
Hydrocyanic acid, aqueous solution, with not more than 20% hydrogen cyanide is a clear colorless aqueous solution of a gas. Has a faint odor of almonds. Can evolve hydrogen cyanide gas, which is (barely) lighter than air. Flame can flash back to the source of a gas leak very easily. Lethal doses of gas may be inhaled. Lethal doses of cyanide can be absorbed from the solution through the skin. Hydrocyanic acid, aqueous solutions, with more than 20% hydrogen cyanide is a clear colorless aqueous solution of a gas. Has an odor of almonds. Can evolve hydrogen cyanide gas, which is (barely) lighter than air. Flame can flash back to the source of a gas leak very easily. Lethal doses of gas may be inhaled. Lethal doses of cyanide can be absorbed from the solution through the skin. Hydrocyanic acid, liquefied appears as a clear colorless liquid with a faint odor of bitter almonds. Boiling point 78°F. Density 5.7 lb / gal. Flash point 0°F. Evaporates easily (or boils) at room temperature. Vapors slightly lighter than air. Deadly poison by all routes (absorption through skin of liquid, inhalation of vapors, etc). Prolonged exposure of closed containers to heat may cause violent rupture and rocketing. Hydrogen cyanide, anhydrous, stabilized appears as very volatile colorless or pale-blue liquid or gas (above 78°F) with a bitter, almond-like odor. Often used as a 96% solution in water. (NIOSH, 2016) A deadly human poison by all routes. Hydrogen cyanide, anhydrous, stabilized (absorbed) appears as a clear colorless liquid with a faint odor of bitter almonds that is absorbed in a porous inert material. Absorption slows evolution of vapors. Vapors slightly lighter than air. Deadly poison by all routes (absorption through skin of liquid, inhalation of vapors, etc). Prolonged exposure of closed containers to heat may cause violent rupture and rocketing. Rate of onset: Immediate Persistence: Minutes Odor threshold: 1-5 ppm Source/use/other hazard: War gas, pesticide, Herbicide; other industries; Weak acid except in water or mucous membranes - then corrosive/training. GasVapor; GasVapor, Liquid; Liquid COLOURLESS GAS OR LIQUID WITH CHARACTERISTIC ODOUR. Colorless or pale-blue liquid or gas (above 78°F) with a bitter, almond-like odor. Colorless or pale-blue liquid or gas (above 78°F) with a bitter, almond-like odor. [Note: Often used as a 96% solution in water.] Colorless or pale blue liquid below 78°F (25.6°C), colorless gas above 78°F (25.6°C). |
ピクトグラム |
Flammable; Acute Toxic; Environmental Hazard |
賞味期限 |
Soln sensitive to light. Anhydrous hydrogen cyanide is stable at or below room temperature if inhibited with acid (eg., 0.1% sulfuric acid). |
溶解性 |
Miscible (NIOSH, 2016) 37.00 M Miscible with water Miscible with ethanol, ethyl ether Miscible with alcohol; slightly soluble in ether Solubility in water: miscible Miscible |
同義語 |
Acid, Hydrocyanic Cyanide, Hydrogen Hydrocyanic Acid Hydrogen Cyanide Zyklon B |
蒸気密度 |
0.901 (EPA, 1998) (Relative to Air) 0.932 (Air = 1) Relative vapor density (air = 1): 0.94 |
蒸気圧 |
630 mm Hg (EPA, 1998) 630 mm Hg (NIOSH, 2016) 741.82 mmHg 742 mm Hg at 25 °C Vapor pressure, kPa at 20 °C: 82.6 630 mmHg |
製品の起源 |
United States |
Synthesis routes and methods I
Procedure details
Synthesis routes and methods II
Procedure details
Synthesis routes and methods III
Procedure details
Retrosynthesis Analysis
AI-Powered Synthesis Planning: Our tool employs the Template_relevance Pistachio, Template_relevance Bkms_metabolic, Template_relevance Pistachio_ringbreaker, Template_relevance Reaxys, Template_relevance Reaxys_biocatalysis model, leveraging a vast database of chemical reactions to predict feasible synthetic routes.
One-Step Synthesis Focus: Specifically designed for one-step synthesis, it provides concise and direct routes for your target compounds, streamlining the synthesis process.
Accurate Predictions: Utilizing the extensive PISTACHIO, BKMS_METABOLIC, PISTACHIO_RINGBREAKER, REAXYS, REAXYS_BIOCATALYSIS database, our tool offers high-accuracy predictions, reflecting the latest in chemical research and data.
Strategy Settings
Precursor scoring | Relevance Heuristic |
---|---|
Min. plausibility | 0.01 |
Model | Template_relevance |
Template Set | Pistachio/Bkms_metabolic/Pistachio_ringbreaker/Reaxys/Reaxys_biocatalysis |
Top-N result to add to graph | 6 |
Feasible Synthetic Routes
試験管内研究製品の免責事項と情報
BenchChemで提示されるすべての記事および製品情報は、情報提供を目的としています。BenchChemで購入可能な製品は、生体外研究のために特別に設計されています。生体外研究は、ラテン語の "in glass" に由来し、生物体の外で行われる実験を指します。これらの製品は医薬品または薬として分類されておらず、FDAから任何の医療状態、病気、または疾患の予防、治療、または治癒のために承認されていません。これらの製品を人間または動物に体内に導入する形態は、法律により厳格に禁止されています。これらのガイドラインに従うことは、研究と実験において法的および倫理的な基準の遵守を確実にするために重要です。