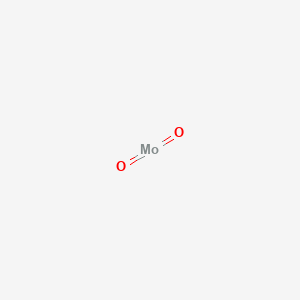
Molybdenum dioxide
説明
Molybdenum dioxide (MoO₂) is a reduced oxide of molybdenum with a monoclinic crystal structure and semi-metallic properties . It exhibits unique electrical conductivity, thermal stability, and electrochemical reversibility, making it a critical material for energy storage, catalysis, and electronics. MoO₂'s oxidation state (+4) distinguishes it from other molybdenum oxides, such as MoO₃ (+6) and mixed-valence suboxides (e.g., Mo₄O₁₁, Mo₈O₂₃) . Its applications span lithium-ion batteries (LIBs), fuel cells, and electrocatalysis due to its ability to facilitate efficient electron transfer and lithium-ion intercalation .
準備方法
Hydrogen Reduction: Industrial and Laboratory-Scale Processes
Industrial Production via Hydrogen Gas Reduction
The industrial synthesis of MoO₂ predominantly involves the reduction of molybdenum trioxide (MoO₃) with hydrogen gas. This two-step process begins with the exothermic reduction of MoO₃ to MoO₂ at 400–700°C, followed by further reduction to metallic molybdenum at higher temperatures . Key parameters include:
-
Feedstock : Pure MoO₃, ammonium heptamolybdate ((NH₄)₆Mo₇O₂₄), or ammonium dimolybdate.
-
Reduction Conditions : Hydrogen flow rates of 4.8–6.7 L/min ensure complete reduction while minimizing intermediate phases like γ-Mo₄O₁₁ .
-
Temperature Dependence : At 500°C, MoO₃ undergoes anisotropic thermal expansion (linear expansion coefficients: αₐ = 1.32 × 10⁻⁴ K⁻¹, α_b = 5.5 × 10⁻⁴ K⁻¹, α_c = −0.246 × 10⁻⁴ K⁻¹), influencing particle morphology .
Table 1: Industrial Hydrogen Reduction Parameters
Parameter | MoO₃ Reduction to MoO₂ | MoO₂ Reduction to Mo |
---|---|---|
Temperature Range | 400–700°C | >700°C |
Hydrogen Flow Rate | 4.8–6.7 L/min | 6–10 L/min |
Reaction Time | 2–4 hours | 3–6 hours |
Key Intermediate | γ-Mo₄O₁₁ | None |
The presence of potassium (0.1–0.5 wt%) accelerates γ-Mo₄O₁₁ formation, which subsequently converts to MoO₂ . This intermediate phase is critical for controlling reaction kinetics and ensuring uniform particle growth.
Laboratory-Scale Hydrogen Reduction
Smaller-scale methods optimize reduction efficiency by modifying reactor design and precursor composition. For instance, patent US20160362303A1 describes a quartz tube reactor (1200 mm length, 30 mm diameter) where MoO₃ powder is reduced at 600°C under nitrogen carrier gas . The process yields polycrystalline MoO₂ with a median particle size of 1–5 µm, suitable for catalytic applications .
Solvothermal and Hydrothermal Synthesis
Aerosol-Assisted Solvothermal Reduction
A breakthrough in nanoparticle synthesis is demonstrated in US20160362303A1 , which combines aerosol pyrolysis with solvothermal reduction . The method involves:
-
Precursor Preparation : Dissolving ammonium molybdate tetrahydrate in distilled water (0.05–0.5 M).
-
Aerosol Generation : Ultrasonic nebulization (1.7 MHz) creates droplets transported via nitrogen gas into a 600°C reactor.
-
Solvothermal Reduction : MoO₃ microparticles are dispersed in ethylene glycol-water (1:3 wt%) and heated at 150–200°C for 10–12 hours .
Ethylene glycol acts as a reducing agent, decomposing to glycolaldehyde (CH₂OHCHO), which facilitates the reduction of Mo⁶⁺ to Mo⁴⁺. This produces MoO₂ nanoparticles (20–50 nm) with high surface area (80–120 m²/g) .
Table 2: Solvothermal Synthesis Conditions and Outcomes
Parameter | Optimal Range | Outcome |
---|---|---|
Precursor Concentration | 0.05–0.5 M | Prevents irregular morphology |
Solvent Ratio (H₂O:EG) | 1:3 | Maximizes MoO₂ yield |
Reaction Temperature | 150–200°C | 20–50 nm nanoparticles |
Reaction Time | 10–12 hours | 99% purity |
Hydrothermal Synthesis of Mixed-Valence Oxides
A sol-gel approach using molybdenum chloro-ethoxide (MoCl₅ + ethanol + K) yields blue amorphous precursors that crystallize into MoO₂ upon vacuum annealing . Residual chlorine (1–6 wt%) is removed by heating to 160°C, while ethoxy groups decompose above 400°C . This method produces mixed-phase oxides (MoO₂, MoO₃, Mo₄O₁₁) under inert atmospheres, highlighting the sensitivity of valence states to reaction conditions.
Sol-Gel and Chemical Precipitation Methods
Peroxomolybdic Acid Organosol Route
Research by RSC Advances details a sol-gel method using peroxomolybdic acid (H₂MoO₆) synthesized via ultrasonic reaction . Annealing at 150°C produces amorphous MoOₓ (x ≈ 2.8–3.0) with a work function of 5.26 eV, ideal for hole-transport layers in solar cells . XPS analysis reveals Mo⁵⁺/Mo⁶⁺ ratios of 1:2 at 150°C, shifting to 1:1 at 200°C due to oxygen vacancy formation .
Table 3: Annealing Effects on MoOₓ Properties
Annealing Temperature | Mo⁵⁺ Fraction | Work Function (eV) | Surface Roughness (nm) |
---|---|---|---|
150°C | 33% | 5.26 | 1.2 |
200°C | 50% | 5.12 | 2.8 |
Chemical Precipitation with Reducing Agents
Early studies employed hydrazine hydrate (N₂H₄·H₂O) to reduce ammonium molybdate in acidic media. This method, though effective, faces challenges in controlling particle size distribution and minimizing residual nitrogen species.
Emerging Techniques and Hybrid Approaches
Plasma-Assisted Reduction
Recent advances utilize hydrogen plasma to reduce MoO₃ at lower temperatures (300–400°C). Plasma excitation enhances hydrogen reactivity, enabling monolayer MoO₂ synthesis for optoelectronic applications.
Mechanochemical Synthesis
Ball-milling MoO₃ with lithium borohydride (LiBH₄) initiates solid-state reduction at room temperature. This technique avoids solvent use but requires post-treatment to remove boron contaminants.
化学反応の分析
Reduction with Hydrogen
The industrial production of MoO₂ involves a two-step hydrogen reduction process :
-
First Step : MoO₃ is reduced to MoO₂ at 450–470°C:
This reaction is exothermic and influenced by hydrogen flow rate and potassium content .
-
Second Step : Further reduction at 850–1050°C produces metallic molybdenum (Mo) .
Reduction with Molybdenum Metal
MoO₂ can also form via self-reduction at 800°C :
Vacuum Thermal Decomposition
MoO₂ forms from MoO₃ in vacuum (1 Pa) without hydrogen at temperatures exceeding 600°C .
Thermal Decomposition
At high temperatures, MoO₂ undergoes decomposition:
Thermodynamic Data :
Property | Value |
---|---|
Melting Point (MoO₂) | 1100°C (decomposition) |
Density | 6.47 g/cm³ |
Heat of Formation | -587.9 kJ/mol |
Oxidation Reactions
MoO₂ acts as an intermediate in oxidation processes:
Reduction Reactions
MoO₂ serves as a catalyst in reduction reactions:
-
Hydrogen Evolution Reaction (HER) : MoO₂ nanoparticles on reduced graphene oxide (rGO) exhibit a low overpotential (190 mV) and Tafel slope (49 mV/decade) in acidic/alkaline media .
-
Lithium-Ion Batteries : MoO₂ shows anomalous lithium storage (1814 mAh/g initial capacity) via Li-ion intercalation and metallic Li-rich phase formation .
Catalytic Reactions
MoO₂’s metallic conductivity and surface activity enable diverse catalytic applications:
-
Dehydrogenation of Alcohols : Converts alcohols to aldehydes/ketones .
-
Hydrocarbon Reformation : Facilitates biodiesel production and methane reformation .
-
Ammoxidation : Transforms hydrocarbons into nitriles and amines in the presence of ammonia .
Reactions with Halogens and Acids
-
Chlorination : MoO₂ reacts with Cl₂ to form molybdenum dichloride dioxide:
-
Stability in Acids : Insoluble in nonoxidizing acids but dissolves in oxidizing agents like HNO₃ .
Reaction Kinetics and Mechanisms
-
MoO₃ Reduction to MoO₂ : Follows a shrinking-core model, with kinetics influenced by hydrogen flow and particle morphology .
-
Decomposition : Fits a two-step 1st-order reaction model, with activation energy derived from thermogravimetric analysis .
Kinetic Parameters for MoO₂ Decomposition :
Step | Activation Energy (kJ/mol) | Frequency Factor (s⁻¹) |
---|---|---|
1 | 120 ± 10 | 1.2 × 10⁸ |
2 | 95 ± 8 | 3.5 × 10⁶ |
Industrial and Environmental Relevance
科学的研究の応用
Energy Storage Applications
Lithium-Ion Batteries
Molybdenum dioxide has emerged as a promising anode material in lithium-ion batteries. Research has demonstrated that mesoporous this compound can achieve a discharge capacity exceeding its theoretical limit, with values reported at 1,814 mAh/g for the first cycle, significantly enhancing the performance of lithium-ion batteries used in electric vehicles and renewable energy storage systems. This high capacity is attributed to its unique electrochemical reaction mechanisms that allow for efficient lithium storage .
Supercapacitors
this compound's high surface area and excellent conductivity make it suitable for supercapacitor applications. Its ability to store charge efficiently contributes to the development of energy storage devices that require rapid charge and discharge cycles, essential for applications in electric vehicles and grid energy storage .
Catalytic Applications
Catalysis in Chemical Reactions
this compound serves as a catalyst in various chemical processes, including the production of acetic acid and other organic compounds. Its catalytic properties facilitate reactions under mild conditions, making it a valuable component in industrial chemistry . Additionally, this compound is utilized in hydrocarbon oxidation processes, enhancing the synthesis of fine chemicals and pharmaceuticals .
Environmental Remediation
The photocatalytic activity of this compound has been explored for environmental applications such as water splitting and pollutant degradation. It can effectively degrade organic pollutants under light irradiation, presenting a sustainable solution for wastewater treatment and environmental cleanup efforts .
Electronic Applications
Semiconductors and Sensors
this compound is employed in the fabrication of electronic components such as semiconductors and gas sensors due to its semiconducting properties. It acts as a hole transport layer in organic solar cells, improving their efficiency by facilitating charge transport within the device . Furthermore, its use in gas sensors allows for the detection of various gases with high sensitivity, making it suitable for environmental monitoring applications .
Thin Films
The development of thin films from this compound has opened avenues for applications in optoelectronics. These films are utilized in devices such as LEDs and displays due to their favorable optical properties and ability to be integrated into flexible electronic systems .
Case Studies
作用機序
The mechanism by which molybdenum dioxide exerts its effects is complex and involves its metallic conductivity and catalytic properties. The delocalization of some of the molybdenum electrons in a conductance band accounts for its metallic conductivity . In catalysis, this compound facilitates the breaking and forming of chemical bonds, thereby accelerating chemical reactions .
類似化合物との比較
Structural and Electronic Properties Comparison
Table 1: Structural and Electronic Properties of Molybdenum Compounds
Compound | Oxidation State | Crystal Structure | Conductivity | Key Applications |
---|---|---|---|---|
MoO₂ | +4 | Monoclinic | Semi-metallic | LIBs, electrocatalysts |
MoO₃ | +6 | Orthorhombic (α) | Insulating | Catalysis, sensors, electrochromics |
MoS₂ | +4 (Mo) | Layered hexagonal | Semiconductor | Nanoelectronics, lubrication |
MoCl₂O₂ | +6 | Tetragonal | Variable | Catalysis, precursor synthesis |
MoO₂-MgSO₄ | Mixed | Composite | Ionic conductor | Thermochemical processing |
- MoO₂ vs. MoO₃ : MoO₂'s semi-metallic conductivity contrasts with MoO₃'s insulating behavior, making the former superior for electrode materials . MoO₃’s layered α-phase is preferred in gas sensing and electrochromic devices due to its wide bandgap (~3 eV) .
- MoO₂ vs. MoS₂: MoS₂’s layered structure enables applications in flexible electronics and catalysis, whereas MoO₂’s dense monoclinic structure supports higher mechanical stability .
- Mixed Oxides : Composites like MoO₂-MgSO₄ (from molybdenite sintering) reduce sulfur dioxide emissions in thermochemical processes .
Thermal and Electrochemical Performance
Table 2: Thermal and Electrochemical Properties
Compound | Melting Point (°C) | Thermal Stability | Electrochemical Capacity (mAh/g) | Cyclic Stability |
---|---|---|---|---|
MoO₂ | ~1,900 | High | ~838 (LIBs) | Excellent |
MoO₃ | ~795 | Moderate | ~1,117 (LIBs, theoretical) | Poor (volume expansion) |
MoS₂ | ~1,185 | High (in inert) | ~670 (LIBs) | Moderate |
MnO₂-MoO₃ | N/A | Moderate | N/A | Improved discharge efficiency |
- LIB Performance : MoO₂ demonstrates superior cyclic stability compared to MoO₃, which suffers from structural degradation during lithium intercalation . MoS₂ offers moderate capacity but excels in high-temperature stability .
- Thermochemical Stability : MoO₂-MgSO₄ composites minimize sulfur emissions during molybdenite decomposition, enhancing industrial sustainability .
Table 3: Catalytic Performance of Molybdenum Compounds
Compound | Catalytic Role | Efficiency Metrics | Key Reactions |
---|---|---|---|
MoO₂ | Electrocatalyst for H₂/O₂ evolution | Overpotential: ~300 mV (HER) | Water splitting, fuel cells |
MoO₃ | Oxidation catalyst | Conversion: >90% (alkene epoxidation) | Petrochemical refining |
MoCl₂O₂ | Precursor for organometallic synthesis | Yield: ~85% (olefin metathesis) | Polymerization, organic synthesis |
MoS₂-SiO₂ | Nanofluid stabilizer | Thermal conductivity: +15% (ethylene glycol) | Heat transfer systems |
- Electrocatalysis: MoO₂ nanoparticles enhance hydrogen evolution reaction (HER) efficiency due to their conductive and defect-rich surfaces .
- Industrial Catalysis: MoO₃ is widely used in alkene epoxidation, while MoCl₂O₂ serves as a versatile precursor for synthesizing organomolybdenum complexes .
- Hybrid Systems: MoS₂-SiO₂ nanofluids improve thermal conductivity in coolants, leveraging synergistic effects between nanoparticles .
生物活性
Molybdenum dioxide (MoO₂) is a transition metal oxide that has garnered attention in various fields, including materials science, catalysis, and biomedical applications. This article delves into the biological activity of MoO₂, focusing on its antimicrobial properties, potential in cancer therapy, and interactions with biological systems.
Antimicrobial Properties
Recent studies have highlighted the antibacterial activity of molybdenum oxides, particularly MoO₃ and its reduced form MoO₂. Research indicates that these compounds exhibit significant antimicrobial effects against various bacterial strains.
- Mechanism of Action : The antibacterial efficacy of MoO₃ and MoO₂ is primarily attributed to the release of hydroxonium ions (H₃O⁺) upon contact with moisture. This process disrupts bacterial cell membranes, leading to DNA damage and the inhibition of protein synthesis, ultimately resulting in cell death .
- Case Study : A study demonstrated that molybdenum oxide nanoparticles effectively reduced contamination by Staphylococcus aureus, a common pathogen responsible for hospital-acquired infections. The study confirmed that the mechanism involved both membrane disruption and the generation of reactive oxygen species (ROS), which further contributed to bacterial cell death .
- Synergistic Effects : When combined with silver nanoparticles, MoO₂ showed enhanced antibacterial properties. This synergy is particularly effective against resistant strains of bacteria such as E. coli and Bacillus subtilis, indicating potential applications in wound dressings and antimicrobial coatings .
Cancer Therapy Applications
This compound has also been explored for its potential in photothermal therapy (PTT) for cancer treatment.
- Nanoparticle Formulation : Bow tie-like MoO₂ nanoparticles have been synthesized to exploit their strong localized surface plasmon resonance (SPR) effects. These nanoparticles demonstrated high stability under biological conditions and exhibited minimal toxicity to normal cells while effectively inhibiting tumor growth under near-infrared laser illumination .
- In Vivo Studies : In vivo experiments revealed that MoO₂ nanoparticles could significantly reduce tumor viability when exposed to near-infrared light, suggesting their utility as a PTT agent. The ability to withstand high temperatures without oxidation further enhances their application in therapeutic settings .
Biocompatibility and Toxicity
The biocompatibility of MoO₂ is an essential factor for its application in biomedical fields. Studies indicate that:
- Low Toxicity : MoO₂ exhibits low toxicity levels in biological environments. In vitro studies have shown negligible cytotoxic effects on healthy cells, making it a promising candidate for drug delivery systems and biomedical imaging .
- Interactions with Biological Systems : The interactions between MoO₂ nanoparticles and immune cells have been investigated, showing that these nanomaterials can modulate immune responses without eliciting significant adverse effects. This characteristic is crucial for developing therapeutic agents that require minimal immune system interference .
Summary Table of Biological Activities
Q & A
Basic Research Questions
Q. What are the standard synthesis methods for producing phase-pure molybdenum dioxide (MoO₂), and how can researchers validate crystallinity and stoichiometry?
- Methodological Answer : MoO₂ is commonly synthesized via solid-state reactions, hydrothermal methods, or mechanochemical approaches. For mechanochemical synthesis, optimize parameters such as precursor molar ratios (e.g., Mo:Fe in MoO₂-hematite composites) and ball-milling duration (e.g., 2–12 hours) to control crystallite size and phase purity . Characterization should include XRD to confirm the monoclinic crystal structure (P2₁/c space group) and Raman spectroscopy to identify Mo-O vibrational modes. Combustion analysis or XPS can verify stoichiometry by quantifying oxygen content .
Q. How do researchers distinguish MoO₂ from other molybdenum oxides (e.g., MoO₃) in spectroscopic data?
- Methodological Answer : MoO₂ exhibits distinct XRD peaks at 2θ ≈ 26.1° (011) and 37.2° (−202), whereas MoO₃ shows orthorhombic phase peaks at 12.8° (020) and 23.3° (110). In XPS, Mo⁴+ in MoO₂ produces a Mo 3d₅/₂ binding energy of ~229 eV, contrasting with Mo⁶+ in MoO₃ (~232 eV). Raman spectra further differentiate via MoO₂’s 460 cm⁻¹ (Mo-O stretching) vs. MoO₃’s 820 cm⁻¹ (terminal Mo=O) .
Advanced Research Questions
Q. What experimental strategies address contradictions in reported thermal stability of MoO₂ under reducing atmospheres?
- Methodological Answer : Discrepancies in decomposition temperatures (e.g., ~1100°C in some studies vs. lower thresholds in others) may arise from impurities or varying partial pressures of oxygen. Use thermogravimetric analysis (TGA) coupled with mass spectrometry (MS) to monitor gas evolution (e.g., O₂ loss) under controlled atmospheres. Compare results with thermodynamic databases (e.g., NIST-JANAF tables) to reconcile experimental and computational ΔHƒ values .
Q. How can edge-site engineering enhance MoO₂’s electrocatalytic activity for reactions like hydrogen evolution (HER) or oxygen reduction (ORR)?
- Methodological Answer : Inspired by MoS₂ studies, MoO₂’s edge sites can be maximized via nanostructuring (e.g., nanoparticles on graphene substrates). Use scanning tunneling microscopy (STM) to quantify edge-site density and correlate it with HER activity via Tafel analysis. For example, a Tafel slope of ~41 mV/decade suggests a Volmer-Heyrovsky mechanism, where edge sites lower the H* adsorption energy .
Q. What computational methods are suitable for modeling MoO₂’s electronic structure and its implications for photoelectrochemical applications?
- Methodological Answer : Density functional theory (DFT) with Hubbard-U corrections (e.g., U = 4–6 eV for Mo 4d orbitals) can accurately predict bandgap (~0.9 eV for MoO₂) and density of states (DOS). Validate computational models with experimental UV-Vis-NIR diffuse reflectance spectra and Hall effect measurements for carrier mobility .
Q. How should researchers resolve discrepancies in reported oxygen vacancy concentrations and their impact on MoO₂’s conductivity?
- Methodological Answer : Combine defect-sensitive techniques:
- Positron annihilation spectroscopy to quantify vacancy density.
- Electron paramagnetic resonance (EPR) to detect unpaired electrons at oxygen vacancy sites.
- Four-point probe measurements to correlate vacancy concentration with electrical conductivity. Cross-reference with ab initio calculations to validate defect formation energies .
Q. Data Analysis & Reproducibility
Q. What statistical approaches are recommended for analyzing heterogeneous catalytic performance data in MoO₂ studies?
- Methodological Answer : Use linear regression to correlate edge-site density (from TEM/STM) with turnover frequency (TOF). For non-normal data distributions (e.g., particle size vs. activity), apply non-parametric tests like Spearman’s rank correlation. Report confidence intervals and effect sizes to quantify uncertainty .
Q. How can researchers ensure reproducibility in MoO₂ synthesis across labs?
- Methodological Answer : Adopt the "supporting information" guidelines from Beilstein Journal of Organic Chemistry:
- Provide raw XRD/TGA data files and detailed synthesis protocols (e.g., milling speed, precursor suppliers).
- For nanoparticles, include dynamic light scattering (DLS) histograms and zeta potential measurements to document colloidal stability .
Q. Ethical & Collaborative Considerations
Q. What guidelines govern the use of shared computational databases (e.g., NIST-JANAF) in MoO₂ thermodynamics research?
- Methodological Answer : Cite primary sources (e.g., Chase, 1998 for NIST-JANAF) and verify data against experimental results. For collaborative projects, define data ownership and accessibility in advance using frameworks like the CRediT taxonomy .
Q. How should interdisciplinary teams allocate tasks in MoO₂-based energy storage projects?
- Methodological Answer : Divide roles using milestones:
- Materials synthesis : Chemists optimize precursors and reaction conditions.
- Device integration : Engineers design electrodes and test cycling stability.
- Data analysis : Statisticians apply machine learning to predict capacity fade.
Document workflows via version-controlled platforms (e.g., GitHub) to ensure transparency .
特性
IUPAC Name |
dioxomolybdenum | |
---|---|---|
Source | PubChem | |
URL | https://pubchem.ncbi.nlm.nih.gov | |
Description | Data deposited in or computed by PubChem | |
InChI |
InChI=1S/Mo.2O | |
Source | PubChem | |
URL | https://pubchem.ncbi.nlm.nih.gov | |
Description | Data deposited in or computed by PubChem | |
InChI Key |
QXYJCZRRLLQGCR-UHFFFAOYSA-N | |
Source | PubChem | |
URL | https://pubchem.ncbi.nlm.nih.gov | |
Description | Data deposited in or computed by PubChem | |
Canonical SMILES |
O=[Mo]=O | |
Source | PubChem | |
URL | https://pubchem.ncbi.nlm.nih.gov | |
Description | Data deposited in or computed by PubChem | |
Molecular Formula |
MoO2 | |
Record name | molybdenum(IV) oxide | |
Source | Wikipedia | |
URL | https://en.wikipedia.org/wiki/Molybdenum(IV)_oxide | |
Description | Chemical information link to Wikipedia. | |
Source | PubChem | |
URL | https://pubchem.ncbi.nlm.nih.gov | |
Description | Data deposited in or computed by PubChem | |
DSSTOX Substance ID |
DTXSID10879986 | |
Record name | Molybdenum dioxide | |
Source | EPA DSSTox | |
URL | https://comptox.epa.gov/dashboard/DTXSID10879986 | |
Description | DSSTox provides a high quality public chemistry resource for supporting improved predictive toxicology. | |
Molecular Weight |
127.95 g/mol | |
Source | PubChem | |
URL | https://pubchem.ncbi.nlm.nih.gov | |
Description | Data deposited in or computed by PubChem | |
Physical Description |
Lead-gray solid; [Hawley] Red-brown odorless powder; Insoluble in water; [MSDSonline] Solid (dark blue crystals) that is a metallic conductor and weakly paramagnetic; [Ullmann] | |
Record name | Molybdenum(IV) oxide | |
Source | Haz-Map, Information on Hazardous Chemicals and Occupational Diseases | |
URL | https://haz-map.com/Agents/8287 | |
Description | Haz-Map® is an occupational health database designed for health and safety professionals and for consumers seeking information about the adverse effects of workplace exposures to chemical and biological agents. | |
Explanation | Copyright (c) 2022 Haz-Map(R). All rights reserved. Unless otherwise indicated, all materials from Haz-Map are copyrighted by Haz-Map(R). No part of these materials, either text or image may be used for any purpose other than for personal use. Therefore, reproduction, modification, storage in a retrieval system or retransmission, in any form or by any means, electronic, mechanical or otherwise, for reasons other than personal use, is strictly prohibited without prior written permission. | |
CAS No. |
18868-43-4 | |
Record name | Molybdenum dioxide | |
Source | CAS Common Chemistry | |
URL | https://commonchemistry.cas.org/detail?cas_rn=18868-43-4 | |
Description | CAS Common Chemistry is an open community resource for accessing chemical information. Nearly 500,000 chemical substances from CAS REGISTRY cover areas of community interest, including common and frequently regulated chemicals, and those relevant to high school and undergraduate chemistry classes. This chemical information, curated by our expert scientists, is provided in alignment with our mission as a division of the American Chemical Society. | |
Explanation | The data from CAS Common Chemistry is provided under a CC-BY-NC 4.0 license, unless otherwise stated. | |
Record name | Molybdenum oxide (MoO2) | |
Source | ChemIDplus | |
URL | https://pubchem.ncbi.nlm.nih.gov/substance/?source=chemidplus&sourceid=0018868434 | |
Description | ChemIDplus is a free, web search system that provides access to the structure and nomenclature authority files used for the identification of chemical substances cited in National Library of Medicine (NLM) databases, including the TOXNET system. | |
Record name | Molybdenum oxide (MoO2) | |
Source | EPA Chemicals under the TSCA | |
URL | https://www.epa.gov/chemicals-under-tsca | |
Description | EPA Chemicals under the Toxic Substances Control Act (TSCA) collection contains information on chemicals and their regulations under TSCA, including non-confidential content from the TSCA Chemical Substance Inventory and Chemical Data Reporting. | |
Record name | Molybdenum dioxide | |
Source | EPA DSSTox | |
URL | https://comptox.epa.gov/dashboard/DTXSID10879986 | |
Description | DSSTox provides a high quality public chemistry resource for supporting improved predictive toxicology. | |
Record name | Molybdenum dioxide | |
Source | European Chemicals Agency (ECHA) | |
URL | https://echa.europa.eu/substance-information/-/substanceinfo/100.038.746 | |
Description | The European Chemicals Agency (ECHA) is an agency of the European Union which is the driving force among regulatory authorities in implementing the EU's groundbreaking chemicals legislation for the benefit of human health and the environment as well as for innovation and competitiveness. | |
Explanation | Use of the information, documents and data from the ECHA website is subject to the terms and conditions of this Legal Notice, and subject to other binding limitations provided for under applicable law, the information, documents and data made available on the ECHA website may be reproduced, distributed and/or used, totally or in part, for non-commercial purposes provided that ECHA is acknowledged as the source: "Source: European Chemicals Agency, http://echa.europa.eu/". Such acknowledgement must be included in each copy of the material. ECHA permits and encourages organisations and individuals to create links to the ECHA website under the following cumulative conditions: Links can only be made to webpages that provide a link to the Legal Notice page. | |
試験管内研究製品の免責事項と情報
BenchChemで提示されるすべての記事および製品情報は、情報提供を目的としています。BenchChemで購入可能な製品は、生体外研究のために特別に設計されています。生体外研究は、ラテン語の "in glass" に由来し、生物体の外で行われる実験を指します。これらの製品は医薬品または薬として分類されておらず、FDAから任何の医療状態、病気、または疾患の予防、治療、または治癒のために承認されていません。これらの製品を人間または動物に体内に導入する形態は、法律により厳格に禁止されています。これらのガイドラインに従うことは、研究と実験において法的および倫理的な基準の遵守を確実にするために重要です。