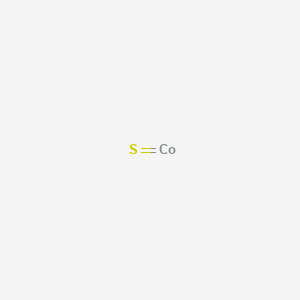
Cobalt sulfide
Vue d'ensemble
Description
Cobalt sulfide (CoS) is a transition metal chalcogenide with multiple stoichiometric phases, including CoS, CoS₂, Co₃S₄, and Co₉S₈, each exhibiting distinct structural, electronic, and catalytic properties . These phases are synthesized via methods such as chemical bath deposition , thermal decomposition of single-source precursors , and solvent-mediated colloidal synthesis . This compound is notable for its high electrical conductivity, redox activity, and mechanical robustness, making it suitable for applications in supercapacitors , lithium-ion batteries , and composite materials . However, synthesis challenges persist, particularly in controlling phase purity and nanostructure morphology, which are influenced by reaction temperature, solvent choice, and sulfur source .
Applications De Recherche Scientifique
Energy Storage
Cobalt sulfide has emerged as a promising material for energy storage devices, particularly in batteries and supercapacitors. Its high conductivity and electrochemical activity make it suitable for these applications.
Key Findings:
- Electrode Materials: CoS nanosheets derived from metal-organic frameworks have shown excellent performance in energy storage devices. These materials exhibit high specific capacitance and stability, making them ideal for supercapacitor applications .
- Battery Applications: this compound composites demonstrate enhanced electrochemical properties when used as anode materials in lithium-ion batteries. The nanosheet structure provides a large surface area for lithium ion intercalation, improving battery efficiency .
Photocatalysis
This compound is recognized for its role as a cocatalyst in photocatalytic reactions. It enhances the efficiency of photocatalysts by facilitating charge separation and transfer.
Applications:
- Hydrogen Production: CoS has been utilized to improve photocatalytic hydrogen evolution reactions. For instance, this compound-modified graphite carbon nitride has been reported to significantly enhance hydrogen production rates due to improved electron migration .
- Pollutant Degradation: this compound-based composites have shown effectiveness in degrading organic pollutants under UV and visible light irradiation. The unique structure of these composites allows for better light absorption and charge separation .
Electrocatalysis
This compound serves as an effective electrocatalyst for various reactions, including oxygen evolution reactions (OER) and hydrogen evolution reactions (HER).
Case Studies:
- Oxygen Evolution Reaction: CoS exhibits a low Tafel slope and high electrochemical activity, making it a suitable candidate for OER applications. Studies have shown that this compound can achieve current densities with minimal overpotential, indicating its potential for water splitting technologies .
- Hydrogen Evolution Reaction: The introduction of this compound into catalytic systems has led to enhanced performance in HER, showcasing its ability to facilitate the necessary redox reactions efficiently .
Biosensing
Recent advancements have highlighted the potential of this compound in biosensing applications, particularly in the detection of biomolecules.
Innovative Applications:
- Biosensors: this compound nanocomposites have been developed for detecting amino acids such as L-arginine. These sensors leverage the high surface area and conductivity of CoS to achieve high sensitivity and selectivity, which can revolutionize molecular diagnostics .
Summary Table of Applications
Mécanisme D'action
Cobalt sulfide exerts its effects primarily through its catalytic properties. In electrocatalysis, this compound facilitates the transfer of electrons during reactions such as the hydrogen evolution reaction and oxygen evolution reaction. The presence of cobalt ions in different oxidation states (Co^2+, Co^3+) allows for efficient electron transfer and adsorption of reaction intermediates, enhancing the overall catalytic performance .
Comparaison Avec Des Composés Similaires
Copper Sulfide (CuS)
Structural and Functional Differences
- Oxidative Behavior : Cobalt sulfide and copper sulfide both form adhesion layers in rubber-brass composites, but cobalt accelerates copper sulfide formation at interfaces, enhancing crosslinking density . Excessive cobalt, however, degrades aged adhesion due to overproduction of copper sulfide .
- Electrochemical Performance : CoS exhibits higher specific capacitance (e.g., 140 m²/g surface area in CoS-HPNs ) compared to CuS, which typically has lower conductivity and stability in supercapacitors.
- Synthesis: Copper sulfide nanoparticles form slowly in montmorillonite clays, whereas CoS synthesis is faster and more solvent-dependent .
Iron Sulfide (FeS)
Challenges in Separation and Processing
- Reductive Smelting : CoS and FeS exhibit similar oxidative-reductive behaviors, leading to co-dissolution in slags during copper-cobalt concentrate processing. This complicates their separation in hydrometallurgical workflows .
- Mechanical Properties : CoS enhances elastic modulus in composites more effectively than FeS. For example, 30 wt% CoS increases elastic modulus to 1746.6 MPa, outperforming FeS-based composites .
Thermal Stability
- CoS phases (e.g., Co₃S₄) decompose at higher temperatures than FeS, enabling stability in high-temperature applications like catalysis .
Molybdenum Sulfide (MoS₂)
Catalytic Synergy
- Hydrotreating Catalysts : CoS-MoS₂ mixtures show superior hydrodesulfurization activity compared to individual sulfides. The Co-Mo bond distances in active phases resemble those in cobalt-molybdenum thiocubane clusters, enhancing catalytic efficiency .
- Electronic Properties : MoS₂ is a semiconductor with a bandgap of ~1.8 eV, while CoS exhibits metallic conductivity, making CoS better suited for conductive composites .
Data Tables
Table 1: Mechanical Properties of this compound Composites
This compound Mass Fraction (wt%) | Elastic Modulus (Experimental, MPa) | Elastic Modulus (FEM Model, MPa) |
---|---|---|
5 | 524.73 | 895.62 |
10 | 944.98 | 991.71 |
20 | 1422.2 | 1484.29 |
30 | 1746.6 | 1784.09 |
Table 2: Electrochemical Performance of CoS-HPNs
Sulfidation Time (h) | Specific Surface Area (m²/g) | Key Morphological Feature |
---|---|---|
1 | 20 | Initial Co-glycerate core |
9 | 140 | Maximum surface area achieved |
24 | 80 | Dense shell, reduced porosity |
Key Research Findings
- Synthesis Optimization: CoS nanoparticles synthesized in ethanol-water solvents exhibit superior cyclic voltammetry performance (specific capacitance ~980 F/g) compared to pure water or ethanol solvents .
- Phase Control: Mixed this compound/oxide nanocomposites in polyphenylenesulfide matrices show tunable optical and magnetic properties, enabling multifunctional applications .
- Aging Effects : Rubber compounds with 0.16 phr cobalt boroacylate retain adhesion strength after thermal and humid aging, outperforming cobalt-free variants .
Activité Biologique
Cobalt sulfide (CoS) is a compound that has garnered attention in various fields, including materials science and biomedicine, due to its unique properties and biological activities. This article reviews the biological activity of this compound, focusing on its antimicrobial effects, potential applications in wound healing, and its role in catalysis.
Antimicrobial Properties
Recent studies have highlighted the antimicrobial properties of this compound nanomaterials. For instance, hollow this compound nanospheres have demonstrated significant peroxidase- and oxidase-like activities , which are crucial for combating bacterial infections. At a concentration of 50 μg/mL, these nanospheres showed effective antibacterial activity against both Gram-positive Staphylococcus aureus and Gram-negative Escherichia coli under physiological conditions .
Table 1: Antibacterial Activity of this compound Nanospheres
Bacterial Strain | Minimum Inhibitory Concentration (MIC) | Activity Observed |
---|---|---|
Staphylococcus aureus | 50 μg/mL | Strong |
Escherichia coli | 50 μg/mL | Strong |
The in vivo studies further confirmed that CoS nanospheres could effectively eliminate bacteria and promote wound healing in infected rats while exhibiting low cytotoxicity . This suggests that this compound could serve as a promising alternative to traditional antibiotics.
The mechanisms underlying the biological activity of this compound involve the generation of reactive oxygen species (ROS), which can induce oxidative stress in microbial cells. The ability of CoS to deplete glutathione (GSH) enhances its enzyme-like properties, contributing to its antimicrobial effects .
Moreover, cobalt compounds have been implicated in various cellular responses, including genotoxicity and the inhibition of DNA repair mechanisms. These effects are thought to be mediated through the release of cobalt ions in vivo, which can interact with cellular components leading to oxidative damage .
Applications in Wound Healing
The application of this compound in wound healing is particularly noteworthy. The nanospheres not only exhibit antibacterial properties but also promote tissue recovery. In studies involving infected rat models, CoS facilitated faster wound closure and reduced bacterial load at the site of infection .
Case Study: In Vivo Wound Healing
A study performed on rats with infected wounds demonstrated that treatment with this compound nanospheres resulted in:
- Significant reduction in bacterial counts.
- Enhanced tissue regeneration observed histologically.
- Low levels of inflammation , indicating good biocompatibility.
Beyond its biological applications, this compound also shows promise as an electrochemical catalyst. It has been reported to catalyze reactions such as the oxygen evolution reaction (OER) and thermocatalytic epoxidation processes. The surface-oxidized forms of CoS exhibit high catalytic activity due to the formation of Co(II) and Co(IV) species during reactions, enhancing its utility in sustainable chemical processes .
Table 2: Catalytic Activities of this compound
Reaction Type | Catalyst Form | Activity Level |
---|---|---|
Oxygen Evolution Reaction (OER) | Surface-oxidized CoS | High |
Epoxidation | This compound | Moderate to High |
Analyse Des Réactions Chimiques
Redox Reactions
CoS participates in redox processes due to cobalt’s variable oxidation states (II, III, IV):
A. Reaction with Nitric Acid
Concentrated nitric acid oxidizes CoS to CoSO₄, releasing NO₂ gas .
B. Hydrochloric Acid and Hydrogen Peroxide
This reaction leverages H₂O₂ to oxidize sulfide ions to elemental sulfur .
Epoxidation of Olefins
CoS catalyzes the epoxidation of cyclooctene using oxidants like PhIO and tBuOOH :
Catalyst | Oxidant | Epoxide Yield (1 h) | Surface Area (m²/g) |
---|---|---|---|
CoSₓ (oxidized) | PhIO | 98% | 35.2 |
Co₃O₄ | PhIO | 42% | 12.8 |
Co(OH)₂ | PhIO | 18% | 8.4 |
Key Findings :
- Oxidized CoS surfaces outperform cobalt oxides due to higher Co(II) density and easier formation of high-valent Co species.
- EPR studies confirm Co(II) and Co(IV) species under reaction conditions, critical for O-transfer .
Oxygen Evolution Reaction (OER)
CoS exhibits electrocatalytic OER activity comparable to IrO₂, with an overpotential of 290 mV at 10 mA/cm² .
Reactions with Acids and Bases
A. Acid Solubility
CoS is insoluble in water but dissolves in concentrated acids:
Solubility Data :
Acid | Solubility (g/100 mL) |
---|---|
HCl | 12.5 |
HNO₃ | 15.8 |
B. Alkaline Stability
CoS reacts with strong bases to form cobalt hydroxides, but stability depends on pH and temperature .
Photocatalytic and Enzyme-Like Activity
- Peroxidase/Oxidase Mimicry: CoS nanospheres (50 μg/mL) catalyze TMB oxidation under physiological pH, with kinetic parameters mM and M/s .
- Hydrogen Production: CoS-based composites show photocatalytic H₂ evolution rates up to 12.3 mmol/g/h under visible light .
Phase Transformations
Thermal treatment induces phase changes:
Temperature (°C) | Phase Formed | Co:S Ratio |
---|---|---|
170 | Amorphous CoS | 1:1 |
220 | Hexagonal Co₉S₈ | 9:8 |
270 | Cubic CoS₂ | 1:2 |
Phase transitions alter catalytic and electronic properties .
Reaction Kinetics
Q & A
Q. Basic: What are the most reliable synthesis methods for cobalt sulfide, and how do experimental parameters influence crystallinity?
This compound can be synthesized via hydrothermal, solvothermal, and spray pyrolysis methods. For hydrothermal synthesis, adjust precursors (e.g., cobalt salts and sulfur sources) and reaction temperatures (typically 120–200°C) to control phase purity . Spray pyrolysis requires precise substrate temperature control (250–400°C) to tune crystallite size and preferred orientations, as demonstrated by XRD patterns showing increased crystallinity at higher temperatures . Always characterize intermediate phases using X-ray diffraction (XRD) to validate stoichiometry.
Q. Basic: How can structural and morphological properties of this compound be systematically characterized?
Use XRD to identify crystallographic phases (e.g., CoS, Co₃S₄, or Co₉S₈) and calculate crystallite size via the Scherrer equation . Complement with scanning electron microscopy (SEM) to analyze surface morphology (e.g., nanowires vs. thin films). Texture coefficients (D, ε, δ) derived from XRD peak intensities quantify preferential growth orientations, as shown in Tables 2–4 for spray-deposited films .
Q. Advanced: What strategies improve the electrochemical stability of this compound in lithium-ion battery anodes?
Encapsulate this compound nanowires in conductive matrices like nitrogen-doped carbon nanotubes (NBNTs) to mitigate volume expansion and enhance conductivity. For example, Co₉S₈@NBNT hybrids exhibit stable capacities (>800 mAh/g after 100 cycles) due to synergistic Li⁺ diffusion pathways and reduced particle aggregation . Optimize carbon-coating thickness via controlled pyrolysis temperatures (500–700°C) during synthesis.
Q. Advanced: How should researchers resolve contradictions in reported electrochemical performance data for this compound?
Conduct comparative studies under standardized testing conditions (e.g., current density, voltage window). Use advanced techniques like X-ray photoelectron spectroscopy (XPS) to assess surface oxidation states and transmission electron microscopy (TEM) to verify nanostructure integrity post-cycling . Cross-reference with computational models (DFT) to explain discrepancies in charge-transfer mechanisms.
Q. Advanced: How does substrate temperature during deposition affect the optical and electronic properties of this compound thin films?
Increasing substrate temperature (250–400°C) enhances crystallinity and reduces lattice strain, as evidenced by narrowed XRD peaks . Optical bandgap (1.7–2.1 eV) can be tuned via temperature-dependent defect states, quantified using Tauc plots from UV-Vis absorption data (Figure 6) . Higher temperatures also improve charge-carrier mobility, critical for photovoltaic applications.
Q. Basic: What methodologies are used to determine the optical bandgap of this compound?
Measure absorption coefficients (α) via UV-Vis spectroscopy and plot (αhν)² vs. photon energy (hν). Extrapolate the linear region to (αhν)² = 0 to obtain the direct bandgap (Figure 6) . For indirect bandgaps, use (αhν)¹/² instead. Account for Urbach tailing in amorphous phases by analyzing absorption edge broadening.
Q. Advanced: How can texture coefficients be applied to quantify crystallographic orientation in this compound thin films?
Calculate texture coefficients , where is the measured XRD peak intensity and is the reference intensity from JCPDS data. Values >1 indicate preferential growth along (hkl) planes. Combine with strain (ε) and dislocation density (δ) calculations to correlate microstructure with optoelectronic properties (Tables 2–4) .
Q. Advanced: What design principles optimize this compound-based electrodes for supercapacitors?
Design 3D hierarchical architectures (e.g., nanosheets on conductive foams) to maximize surface area and ion accessibility. Use cyclic voltammetry (CV) and electrochemical impedance spectroscopy (EIS) to evaluate pseudocapacitive contributions vs. double-layer capacitance. Doping with heteroatoms (e.g., N, P) can enhance conductivity and redox activity .
Q. Basic: What advanced characterization techniques are critical for studying this compound’s surface chemistry?
XPS for oxidation state analysis (Co²⁺ vs. Co³⁺, S²⁻ vs. S⁻), TEM for nanostructure imaging, and in-situ XRD to monitor phase transitions during lithiation/delithiation . Pair with Raman spectroscopy to identify vibrational modes of sulfur bonds (e.g., S-S vs. metal-sulfur).
Q. Advanced: How can researchers ensure reproducibility in this compound synthesis?
Document synthesis parameters exhaustively: precursor molar ratios, reaction time/temperature, and post-treatment steps (e.g., annealing atmosphere). Use factorial experimental designs (e.g., varying pH, temperature, and concentration) to identify critical variables . Share raw data (XRD, SEM) in supplementary materials and adhere to reporting standards (e.g., Beilstein Journal guidelines) .
Méthodes De Préparation
Solution-Based Synthesis and Phase Engineering
Thiourea-Mediated Kinetic Control of Cobalt Sulfide Phases
A breakthrough in phase-selective synthesis was achieved through the use of substituted thioureas as sulfur precursors, which modulate decomposition kinetics to favor specific this compound phases . By varying the substituents on thiourea (e.g., phenyl vs. ethyl groups), researchers demonstrated control over sulfur release rates, enabling selective crystallization of metastable phases. For instance:
-
Fast-decomposing thioureas (e.g., diphenylthiourea) produce high sulfur chemical potentials, favoring cubic close-packed (ccp) cattierite (CoS) at low temperatures (180–220°C) .
-
Slow-decomposing analogs (e.g., diethylthiourea) permit intermediate sulfur concentrations, stabilizing ccp linnaeite (CoS) and hexagonal close-packed (hcp) jaipurite (CoS) at higher temperatures (220–270°C) .
Critical to this approach is the relationship between sulfur precursor stoichiometry and phase evolution. A 6:1 sulfur-to-cobalt ratio promotes linnaeite formation, while reducing this ratio to 1:1 shifts the equilibrium toward cobalt pentlandite (CoS) by limiting sulfur availability for phase transformations . Time-resolved X-ray diffraction studies revealed that CoS acts as a metastable intermediate, transforming into CoS through sulfur incorporation into the ccp lattice over 1–2 hours at 220°C .
Hollow Nanosphere Synthesis via Carbon Disulfide Modulation
Hollow nanostructures of CoS with tunable composition were synthesized using a carbon disulfide (CS)-mediated solvothermal method . By adjusting the CS:Co(OAc) molar ratio from 1:1 to 4:1, phase-pure CoS, CoS, and CoS hollow nanospheres (HNSs) were obtained (Table 1).
Table 1: Phase Control in CoS Hollow Nanospheres via CS Stoichiometry
CS:Co Ratio | Dominant Phase | Diameter (nm) | Shell Thickness (nm) |
---|---|---|---|
1:1 | CoS | 180 ± 20 | 25 ± 5 |
2:1 | CoS | 200 ± 25 | 30 ± 5 |
4:1 | CoS | 220 ± 30 | 35 ± 5 |
The hollow morphology arises from Ostwald ripening, where initial solid nanoparticles undergo preferential dissolution at the core while recrystallizing at the surface . This method’s scalability was demonstrated through gram-scale syntheses, with CoS HNSs exhibiting exceptional electrocatalytic activity for overall water splitting (1.54 V cell voltage at 10 mA cm) .
Vapor-Phase Deposition of 2D this compound Sheets
Epitaxial Growth on Au(111) Substrates
Ultra-thin 2D CoS sheets were synthesized via cobalt evaporation in a HS atmosphere (2 × 10 mbar) onto Au(111) substrates held at 410°C . Post-deposition annealing at 650°C for 20 minutes followed by controlled cooling produced metallic CoS(0001)-like sheets with a lattice constant of 3.16 Å, matching density functional theory (DFT) predictions . Scanning tunneling microscopy (STM) revealed a honeycomb structure with Co atoms occupying threefold hollow sites of the sulfur sublattice (Figure 1A).
Figure 1: (A) STM image of 2D CoS on Au(111) (5 × 5 nm), (B) LEED pattern showing (√3 × √3)R30° reconstruction
X-ray photoelectron spectroscopy (XPS) confirmed a Co:S stoichiometry of 1:1.05, consistent with jaipurite (CoS) . The metallic character of these sheets, attributed to partially filled Co 3d orbitals, makes them promising for quantum confinement applications.
Phase-Pure Synthesis Strategies
Seeded Growth for Linnaeite (Co3_33S4_44) Crystallization
Phase-pure linnaeite was achieved through a two-step seeded approach :
-
Seed synthesis : CoS nanoparticles (5–8 nm) were prepared using 1:1 Co:S stoichiometry at 220°C.
-
Sulfur infusion : Seeds reacted with 6 mmol diphenylthiourea at 270°C, enabling diffusion-controlled transformation to CoS.
This method bypassed hcp jaipurite co-nucleation by exploiting the immiscibility of ccp and hcp phases under reducing conditions . The resulting linnaeite exhibited sheet-like morphologies (20–50 nm lateral dimensions) analogous to greigite (FeS) .
Thermodynamic Stabilization of Jaipurite (CoS)
Phase-pure CoS required a one-pot heat-up method with 18 equivalents of diethylthiourea at 155°C . Excess sulfur precursor suppressed ccp phase nucleation while promoting octahedral Co coordination in hcp jaipurite. Transmission electron microscopy (TEM) showed 5–15 nm crystallites with 9 nm coherence length from Scherrer analysis .
Comparative Analysis of Synthesis Methods
Table 2: Synthesis Techniques and Phase Outcomes
Electrocatalytic Performance of this compound Phases
CoS hollow nanospheres demonstrated superior bifunctional activity in 1 M KOH :
-
OER : Overpotential = 290 mV @ 10 mA cm, Tafel slope = 57 mV dec
-
HER : Overpotential = 193 mV @ 10 mA cm, Tafel slope = 100 mV dec
This outperformed CoS (OER: 320 mV) and CoS (HER: 210 mV), attributed to CoS's higher sulfur content enhancing active site exposure .
Propriétés
Numéro CAS |
1317-42-6 |
---|---|
Formule moléculaire |
CoS |
Poids moléculaire |
91.00 g/mol |
Nom IUPAC |
cobalt(2+);sulfide |
InChI |
InChI=1S/Co.S/q+2;-2 |
Clé InChI |
INPLXZPZQSLHBR-UHFFFAOYSA-N |
SMILES |
S=[Co] |
SMILES canonique |
[S-2].[Co+2] |
Color/Form |
Black amorphous powde |
Densité |
5.45 g/cu cm 5.5 g/cm³ |
melting_point |
1117 °C >1116 °C |
Key on ui other cas no. |
1317-42-6 |
Description physique |
OtherSolid; PelletsLargeCrystals GREY POWDER OR REDDISH-SILVERY CRYSTALS. |
Pictogrammes |
Irritant; Environmental Hazard |
Numéros CAS associés |
1317-42-6 (Parent) |
Solubilité |
Insoluble in water 0.00038 g/100 cc H2O at 18 °C Soluble in acid Solubility in water: none |
Synonymes |
cobalt sulfide cobalt sulphide cobaltous disulfide cobaltous sulfide jaipurite |
Origine du produit |
United States |
Avertissement et informations sur les produits de recherche in vitro
Veuillez noter que tous les articles et informations sur les produits présentés sur BenchChem sont destinés uniquement à des fins informatives. Les produits disponibles à l'achat sur BenchChem sont spécifiquement conçus pour des études in vitro, qui sont réalisées en dehors des organismes vivants. Les études in vitro, dérivées du terme latin "in verre", impliquent des expériences réalisées dans des environnements de laboratoire contrôlés à l'aide de cellules ou de tissus. Il est important de noter que ces produits ne sont pas classés comme médicaments et n'ont pas reçu l'approbation de la FDA pour la prévention, le traitement ou la guérison de toute condition médicale, affection ou maladie. Nous devons souligner que toute forme d'introduction corporelle de ces produits chez les humains ou les animaux est strictement interdite par la loi. Il est essentiel de respecter ces directives pour assurer la conformité aux normes légales et éthiques en matière de recherche et d'expérimentation.