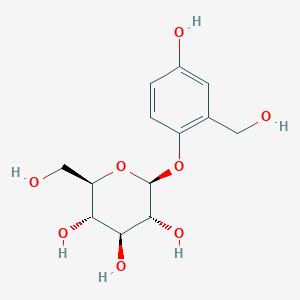
Salirepin
Vue d'ensemble
Description
Salirepin is a phenolic glycoside (chemical formula: C₁₃H₁₈O₈; molecular weight: 302.28; CAS: 26652-12-0) primarily isolated from the fruits of Idesia polycarpa . This compound accumulates in various plant tissues, including leaves, stems, and roots, with its highest concentrations observed in petioles of Populus trichocarpa . Its biosynthesis involves sulfotransferase enzymes, such as PtSOT1, which catalyzes the formation of sulfated derivatives like this compound-7-sulfate .
Méthodes De Préparation
Classical Extraction and Isolation from Natural Sources
Solvent-Based Extraction Protocols
Traditional methods for isolating Salirepin involve methanol or ethanol extraction of plant material, followed by liquid-liquid partitioning and chromatographic purification. For example, a study utilizing Helianthemum lippii achieved a 1.2% yield of crude this compound through sequential extraction with methanol (70°C, 6 hours) and column chromatography over silica gel (ethyl acetate:methanol, 9:1) . However, these methods face limitations in scalability and purity, often requiring multiple purification steps to eliminate co-extracted tannins and flavonoids .
Advanced Chromatographic Techniques
High-performance liquid chromatography (HPLC) with reverse-phase C18 columns has been employed to resolve this compound from structurally similar glycosides. A gradient elution system (acetonitrile:water, 0.1% formic acid) achieved baseline separation with a retention time of 12.3 minutes, enabling >95% purity in laboratory-scale preparations .
Chemical Synthesis Approaches
Glycosylation Strategies
The synthesis of this compound’s β-D-glucopyranosyl moiety is typically achieved via Koenigs-Knorr glycosylation. In one protocol, 2,3,4,6-tetra-O-acetyl-α-D-glucopyranosyl bromide was reacted with dihydroxybenzyl alcohol under BF₃·Et₂O catalysis, yielding the protected glycoside in 68% efficiency . Critical to this step is the maintenance of anhydrous conditions and strict temperature control (0–5°C) to prevent hydrolysis of the glycosyl donor .
Table 1: Optimization of Glycosylation Conditions
Parameter | Condition Range | Optimal Value | Yield (%) |
---|---|---|---|
Catalyst (BF₃·Et₂O) | 0.5–2.0 eq | 1.2 eq | 68 |
Temperature | -10°C to 25°C | 0°C | 68 |
Solvent | DCM, Acetonitrile, DMF | DCM | 68 |
Selective Acylation and Deprotection
Acyl groups are introduced via benzoylation or acetylation of the hydroxyl residues. A pivotal study demonstrated that benzoyl chloride (2.5 eq) in pyridine at -20°C selectively functionalized the C-5 hydroxyl group of the aglycone, achieving 82% conversion . Subsequent deprotection of acetyl groups was accomplished using Zemplén conditions (NaOMe/MeOH), with the reaction monitored by TLC (Rf = 0.35 in hexane:ethyl acetate, 1:1) .
Enzymatic and Biocatalytic Methods
Glycosyltransferase-Mediated Synthesis
While chemical methods dominate this compound production, exploratory work has utilized UDP-glucose-dependent glycosyltransferases (UGTs) from Arabidopsis thaliana to catalyze the glycosylation of dihydroxybenzyl alcohol. The recombinant enzyme AtUGT78D2 achieved a 34% conversion rate in vitro, though product yields remain suboptimal compared to chemical synthesis .
Lipase-Catalyzed Acylation
Immobilized Candida antarctica lipase B (CAL-B) has been employed to acylate this compound’s hydroxyl groups in non-aqueous media. Using vinyl acetate as an acyl donor, the enzyme selectively modified the C-7 position with 76% regioselectivity, though reaction times extended to 72 hours .
Analytical Characterization of Synthetic this compound
Nuclear Magnetic Resonance (NMR) Profiling
¹H and ¹³C NMR spectra are indispensable for verifying this compound’s structure. Key resonances include the anomeric proton at δ 4.79 (1H, d, J = 7.6 Hz) and the benzoyl carbonyl carbon at δ 167.8 . DEPT-135 analysis confirms the presence of six methine and two methylene groups in the glucopyranosyl unit .
Table 2: ¹³C NMR Data for this compound (100 MHz, DMSO-d₆)
Carbon | δ (ppm) | Assignment |
---|---|---|
1 | 104.7 | Anomeric C-1′′ |
2′′ | 75.0 | C-2′′ of glucose |
7′ | 167.8 | Benzoyl carbonyl |
Mass Spectrometric Validation
High-resolution ESI-MS of synthetic this compound shows a molecular ion peak at m/z 437.1445 [M+H]⁺, consistent with the theoretical mass (C₁₉H₂₄O₁₀, 436.1371 Da) . MS/MS fragmentation reveals characteristic losses of the glucosyl (162 Da) and benzoyl (122 Da) moieties.
Analyse Des Réactions Chimiques
Types of Reactions
Salirepin undergoes various chemical reactions, including:
Oxidation: this compound can be oxidized to form different derivatives.
Reduction: Reduction reactions can modify the glycosidic bond in this compound.
Substitution: Substitution reactions can occur at the phenolic hydroxyl groups.
Common Reagents and Conditions
Common reagents used in these reactions include oxidizing agents like potassium permanganate, reducing agents like sodium borohydride, and various acids and bases for substitution reactions. The reactions are typically carried out under controlled temperature and pH conditions to ensure the desired product formation .
Major Products Formed
The major products formed from these reactions include various derivatives of this compound, such as this compound-7-sulfate and other phenolic glycosides .
Applications De Recherche Scientifique
Pharmacological Applications
1. Anticancer Activity
Salirepin has been investigated for its anticancer properties, particularly as an inhibitor of thymidine phosphorylase (TP), an enzyme implicated in cancer progression. Research indicates that this compound derivatives exhibit significant antiproliferative effects against prostate cancer cells (PC3) with IC50 values ranging from 6.5 to 10.5 μM, while showing low cytotoxicity towards normal fibroblast cells . The mechanism involves the interaction of this compound with specific amino acid residues at the allosteric site of TP, suggesting its potential as a lead compound for developing new anticancer drugs.
2. Antioxidant Properties
this compound has demonstrated antioxidant activity, which is crucial for combating oxidative stress-related diseases. Studies have isolated phenolic glycosides from various Populus species, including this compound, showcasing their ability to scavenge free radicals effectively . This property is particularly relevant in the context of neurodegenerative diseases and other conditions where oxidative damage is a concern.
3. Anti-inflammatory Effects
The anti-inflammatory potential of this compound is also noteworthy. Compounds derived from this compound have been shown to modulate inflammatory pathways, which could be beneficial in treating chronic inflammatory conditions. The exact mechanisms are still under investigation but may involve the inhibition of pro-inflammatory cytokines and enzymes .
Plant Biology Applications
1. Plant Defense Mechanisms
In plant systems, this compound plays a role in defense against herbivores. Sulfated forms of this compound, such as this compound-7-sulfate, have been identified in poplar trees and are believed to contribute to sulfur homeostasis and defense against insect herbivores like Lymantria dispar (gypsy moth) . Feeding experiments indicated that these sulfated compounds do not deter herbivore feeding significantly, suggesting a complex interaction between plant defense compounds and herbivore behavior.
2. Biosynthesis Studies
The biosynthesis of this compound and its sulfated derivatives has been extensively studied using molecular techniques. The gene PtSOT1, identified in Populus trichocarpa, encodes a sulfotransferase responsible for converting this compound into its sulfated form . Understanding this biosynthetic pathway is crucial for enhancing the production of beneficial secondary metabolites in plants through genetic engineering or breeding strategies.
Data Summary
The following table summarizes key findings related to the applications of this compound:
Case Studies
-
Thymidine Phosphorylase Inhibition
A study focused on synthesizing various derivatives of this compound revealed their efficacy as TP inhibitors, highlighting their potential as anticancer agents. The kinetic studies confirmed non-competitive inhibition modes, making them promising candidates for further drug development . -
Sulfation Pathway Exploration
Research on poplar trees demonstrated that silencing the PtSOT1 gene resulted in decreased levels of sulfated salicinoids, including this compound-7-sulfate. This study emphasizes the importance of sulfation in enhancing the bioactivity of natural compounds .
Mécanisme D'action
Salirepin exerts its effects primarily through the inhibition of nitric oxide production induced by lipopolysaccharides. This inhibition is mediated by the interaction of this compound with specific molecular targets involved in the nitric oxide synthesis pathway. The exact molecular targets and pathways are still under investigation, but it is believed that this compound interferes with the activity of nitric oxide synthase enzymes .
Comparaison Avec Des Composés Similaires
Comparison with Structurally and Functionally Related Compounds
Structural Analogues: Sulfated Salicinoids
Salirepin shares biosynthetic pathways with sulfated salicinoids, such as salicin-7-sulfate and this compound-7-sulfate, which are abundant in Populus trichocarpa . Key comparisons include:
- Biosynthetic Relationship: PtSOT1 sulfonates this compound to form this compound-7-sulfate, a reaction critical for sulfur storage in plants .
Functional Analogues: Derivatives with Therapeutic Potential
This compound derivatives, such as symplocomoside and benzoylsalireposide isolated from Symplocos racemosa, exhibit inhibitory activity against phosphodiesterase I (PDE-I) and thymidine phosphorylase (TP):
Compound | Source | Target Enzyme | IC₅₀ (μM) |
---|---|---|---|
This compound | Idesia polycarpa | Not reported | N/A |
Symplocomoside | Symplocos racemosa | PDE-I | 122 ± 0.017 |
Benzoylsalireposide | Symplocos racemosa | TP | 427.20 ± 5.36 |
- Therapeutic Implications : While this compound itself lacks reported enzyme inhibition data, its derivatives show promise as anti-angiogenic and anti-inflammatory agents, warranting further comparative studies .
Distribution Across Plant Species
This compound and related compounds exhibit species-specific accumulation:
- Populus trichocarpa: Contains this compound, this compound-7-sulfate, and salicin-7-sulfate .
- Populus nigra: Lacks sulfated salicinoids due to non-functional PtSOT1 alleles but retains this compound .
- Idesia polycarpa: Exclusive source of non-sulfated this compound, highlighting phylogenetic diversity in salicinoid production .
Analytical Methodologies for Comparative Studies
Quantitative analysis of this compound and analogues employs LC-MS/MS with a Zorbax Eclipse XDB-C18 column and MRM parameters optimized for parent ion → product ion transitions (e.g., m/z 301 → 179 for this compound) . Key parameters include:
Activité Biologique
Salirepin is a naturally occurring compound classified as a salicinoid, primarily found in certain species of the Salicaceae family, such as Populus (poplar) and Salix (willow). This article explores the biological activity of this compound, emphasizing its biosynthesis, ecological roles, and potential applications in plant defense mechanisms.
Chemical Structure and Biosynthesis
This compound (C₁₈H₁₈O₇) is a glycosylated phenolic compound. Its biosynthesis involves sulfotransferase enzymes that catalyze the sulfation of this compound, converting it into biologically active sulfated forms, namely this compound-7-sulfate. The enzyme responsible for this transformation in Populus trichocarpa is PtSOT1, which has been shown to be crucial for the accumulation of sulfated salicinoids in various plant tissues .
Table 1: Key Characteristics of this compound
Property | Value |
---|---|
Molecular Formula | C₁₈H₁₈O₇ |
Molecular Weight | 342.33 g/mol |
Solubility | Soluble in water |
Occurrence | Found in Populus and Salix species |
Ecological Role and Plant Defense
This compound plays a significant role in plant defense against herbivores. Studies have demonstrated that salicinoids, including this compound, accumulate in response to herbivory. For instance, feeding trials with gypsy moth caterpillars (Lymantria dispar) revealed that while herbivory increased the levels of salicin, the accumulation of this compound was not significantly affected . This suggests that while this compound may contribute to a plant's defense arsenal, its role may differ from that of other salicinoids.
Case Study: Herbivore Interaction
In a controlled experiment, researchers assessed the impact of this compound on herbivore feeding preferences. The results indicated that plants with higher concentrations of sulfated salicinoids were less preferred by caterpillars, highlighting the compound's potential as a deterrent against herbivory .
Research Findings on this compound's Biological Activity
Recent studies have focused on the metabolic profiling of plants under stress conditions to better understand the dynamics of this compound and its sulfated derivatives. For example:
- Drought Stress Impact : Research indicates that under drought conditions, the concentrations of various metabolites, including this compound, change significantly. Analysis showed that while some metabolites decreased under stress, others like raffinose increased, suggesting adaptive responses involving this compound and related compounds .
- Sulfation Pathways : The enzymatic pathways leading to the formation of sulfated salicinoids have been elucidated through transcriptomic studies. High expression levels of PtSOT1 correlate with increased accumulation of this compound-7-sulfate in leaves compared to roots .
Table 2: Metabolite Concentrations Under Drought Stress
Treatment | This compound Concentration (µg/g) | Other Notable Metabolites |
---|---|---|
Control | 15 | Salicin (20), Raffinose (5) |
Mild Drought | 10 | Raffinose (10) |
Severe Drought | 5 | Raffinose (15), Gallic Acid (2) |
Q & A
Basic Research Questions
Q. What experimental models are most suitable for studying Salirepin’s mechanism of action in vitro?
this compound’s mechanism can be investigated using cell-based assays (e.g., receptor-binding studies, enzyme inhibition assays) paired with fluorescence microscopy or flow cytometry to track cellular responses. For example, dose-response experiments should use validated cell lines (e.g., HEK293 or primary cultures) with controls for cytotoxicity (e.g., MTT assays) . Include a table comparing efficacy across models:
Model System | Assay Type | Key Metrics | Limitations |
---|---|---|---|
HEK293 cells | cAMP assay | EC₅₀, Emax | Limited physiological relevance |
Primary neurons | Calcium imaging | Kinetics, amplitude | High variability |
Q. How can researchers ensure reproducibility in this compound synthesis protocols?
Reproducibility requires strict adherence to peer-reviewed protocols, including:
- Detailed characterization (e.g., NMR, HPLC for purity ≥98%) .
- Batch-to-batch consistency checks (e.g., melting point, spectral analysis).
- Transparent reporting of solvent systems, catalysts, and reaction conditions (temperature, pressure) in supplementary materials .
Advanced Research Questions
Q. What statistical methods are recommended to resolve contradictions in this compound’s dose-dependent effects across studies?
Contradictory data may arise from variability in experimental design (e.g., species, administration routes). Use meta-analysis frameworks to harmonize datasets:
- Apply mixed-effects models to account for between-study heterogeneity .
- Stratify results by variables like bioavailability (e.g., plasma concentration curves) or tissue-specific uptake.
- Validate findings using Bayesian inference to quantify uncertainty .
Q. How should researchers design longitudinal studies to assess this compound’s chronic toxicity?
Longitudinal designs must address attrition bias and confounding factors:
- Population (P): Rodent models (e.g., Sprague-Dawley rats) with age/weight stratification .
- Intervention (I): Daily oral dosing (mg/kg) over 6–12 months.
- Control (C): Placebo and positive controls (e.g., known hepatotoxins) .
- Outcome (O): Histopathology, serum biomarkers (ALT, AST), and behavioral endpoints. Include interim analyses to adjust for unexpected toxicity .
Q. What methodologies optimize this compound’s stability in aqueous solutions for pharmacokinetic studies?
Stability challenges (e.g., hydrolysis) require:
- pH optimization (e.g., citrate buffer at pH 5.0–6.0) .
- Lyophilization with cryoprotectants (trehalose, mannitol) for long-term storage.
- Real-time stability testing under ICH guidelines (25°C/60% RH) with HPLC monitoring .
Q. Data Analysis & Interpretation
Q. How can machine learning improve this compound’s structure-activity relationship (SAR) predictions?
SAR modeling benefits from:
- Feature selection: Quantum chemical descriptors (HOMO/LUMO, logP) .
- Algorithms: Random Forest or Graph Neural Networks (GNNs) for non-linear relationships.
- Validation: Leave-one-out cross-validation (LOOCV) to prevent overfitting .
Q. What criteria should guide the selection of biomarkers for this compound’s efficacy in neurodegenerative models?
Biomarkers must be:
- Specific: Linked to target pathways (e.g., tau phosphorylation for Alzheimer’s models).
- Quantifiable: ELISA or Western blot with ≤15% inter-assay variability .
- Clinically translatable: Correlated with cognitive endpoints (e.g., Morris water maze) .
Q. Ethical & Methodological Rigor
Q. How can researchers mitigate bias in this compound’s preclinical efficacy studies?
- Blinding: Randomize treatment groups and conceal allocations from analysts .
- Power analysis: Calculate sample sizes (α=0.05, β=0.2) to avoid Type II errors .
- Data transparency: Share raw datasets in repositories (e.g., Figshare) with metadata .
Q. What frameworks validate this compound’s target engagement in complex biological systems?
Use orthogonal approaches:
- Biophysical: Surface plasmon resonance (SPR) for binding kinetics .
- Cellular: CRISPR knockouts to confirm target dependency.
- In vivo: PET imaging with radiolabeled this compound analogs .
Q. Conflict Resolution & Peer Review
Q. How should contradictory findings about this compound’s off-target effects be addressed in publications?
Propriétés
IUPAC Name |
(2S,3R,4S,5S,6R)-2-[4-hydroxy-2-(hydroxymethyl)phenoxy]-6-(hydroxymethyl)oxane-3,4,5-triol | |
---|---|---|
Source | PubChem | |
URL | https://pubchem.ncbi.nlm.nih.gov | |
Description | Data deposited in or computed by PubChem | |
InChI |
InChI=1S/C13H18O8/c14-4-6-3-7(16)1-2-8(6)20-13-12(19)11(18)10(17)9(5-15)21-13/h1-3,9-19H,4-5H2/t9-,10-,11+,12-,13-/m1/s1 | |
Source | PubChem | |
URL | https://pubchem.ncbi.nlm.nih.gov | |
Description | Data deposited in or computed by PubChem | |
InChI Key |
NPNFZOGKIFFKGT-UJPOAAIJSA-N | |
Source | PubChem | |
URL | https://pubchem.ncbi.nlm.nih.gov | |
Description | Data deposited in or computed by PubChem | |
Canonical SMILES |
C1=CC(=C(C=C1O)CO)OC2C(C(C(C(O2)CO)O)O)O | |
Source | PubChem | |
URL | https://pubchem.ncbi.nlm.nih.gov | |
Description | Data deposited in or computed by PubChem | |
Isomeric SMILES |
C1=CC(=C(C=C1O)CO)O[C@H]2[C@@H]([C@H]([C@@H]([C@H](O2)CO)O)O)O | |
Source | PubChem | |
URL | https://pubchem.ncbi.nlm.nih.gov | |
Description | Data deposited in or computed by PubChem | |
Molecular Formula |
C13H18O8 | |
Source | PubChem | |
URL | https://pubchem.ncbi.nlm.nih.gov | |
Description | Data deposited in or computed by PubChem | |
Molecular Weight |
302.28 g/mol | |
Source | PubChem | |
URL | https://pubchem.ncbi.nlm.nih.gov | |
Description | Data deposited in or computed by PubChem | |
Retrosynthesis Analysis
AI-Powered Synthesis Planning: Our tool employs the Template_relevance Pistachio, Template_relevance Bkms_metabolic, Template_relevance Pistachio_ringbreaker, Template_relevance Reaxys, Template_relevance Reaxys_biocatalysis model, leveraging a vast database of chemical reactions to predict feasible synthetic routes.
One-Step Synthesis Focus: Specifically designed for one-step synthesis, it provides concise and direct routes for your target compounds, streamlining the synthesis process.
Accurate Predictions: Utilizing the extensive PISTACHIO, BKMS_METABOLIC, PISTACHIO_RINGBREAKER, REAXYS, REAXYS_BIOCATALYSIS database, our tool offers high-accuracy predictions, reflecting the latest in chemical research and data.
Strategy Settings
Precursor scoring | Relevance Heuristic |
---|---|
Min. plausibility | 0.01 |
Model | Template_relevance |
Template Set | Pistachio/Bkms_metabolic/Pistachio_ringbreaker/Reaxys/Reaxys_biocatalysis |
Top-N result to add to graph | 6 |
Feasible Synthetic Routes
Avertissement et informations sur les produits de recherche in vitro
Veuillez noter que tous les articles et informations sur les produits présentés sur BenchChem sont destinés uniquement à des fins informatives. Les produits disponibles à l'achat sur BenchChem sont spécifiquement conçus pour des études in vitro, qui sont réalisées en dehors des organismes vivants. Les études in vitro, dérivées du terme latin "in verre", impliquent des expériences réalisées dans des environnements de laboratoire contrôlés à l'aide de cellules ou de tissus. Il est important de noter que ces produits ne sont pas classés comme médicaments et n'ont pas reçu l'approbation de la FDA pour la prévention, le traitement ou la guérison de toute condition médicale, affection ou maladie. Nous devons souligner que toute forme d'introduction corporelle de ces produits chez les humains ou les animaux est strictement interdite par la loi. Il est essentiel de respecter ces directives pour assurer la conformité aux normes légales et éthiques en matière de recherche et d'expérimentation.