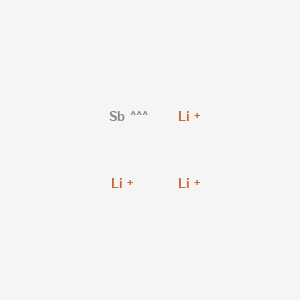
Lithium antimonide
Descripción general
Descripción
Lithium antimonide (SbLi₃) is an intermetallic compound synthesized through electrolysis of a mixture of lithium chloride (LiCl) and potassium chloride (KCl) using an antimony (Sb) cathode, as first reported by M. P. Lebeau . Direct combination of lithium and antimony is highly exothermic but often yields non-stoichiometric products due to the reaction's violent nature. The compound crystallizes in a structure that remains unspecified in available literature but is noted for its low fusibility compared to its constituent elements and strong reducing properties .
Its electrochemical stability and reversible lithium-ion insertion/extraction mechanisms make it a candidate for anode materials . Additionally, it forms a coordination complex with liquid ammonia (SbLi₃·NH₃), which exhibits distinct solubility and reactivity .
Métodos De Preparación
Synthetic Routes and Reaction Conditions: Lithium antimonide can be synthesized through several methods. One common approach involves the reaction of lithium with antimony in anhydrous liquid ammonia. The process begins with the preparation of a suspension of freshly powdered antimony in anhydrous liquid ammonia. A solution of lithium in dried liquid ammonia is then added to the suspension in small portions. The reaction initiates upon shaking, and its completion is indicated by the appearance of a blue color in the supernatant fluid, due to a slight excess of lithium. The ammonia is then boiled out, and the last traces are removed by vacuum, resulting in the formation of this compound powder .
Industrial Production Methods: In industrial settings, this compound can be produced by heating a mixture of lithium and antimony at high temperatures. The mixture is typically heated in a thick-walled iron crucible with a welded-on lid to prevent oxidation. The heating process is carried out at temperatures between 1150°C and 1300°C, followed by quenching under an inert atmosphere such as argon .
Análisis De Reacciones Químicas
Types of Reactions: Lithium antimonide undergoes various chemical reactions, including oxidation, reduction, and intercalation.
Common Reagents and Conditions:
Oxidation: this compound can be oxidized in the presence of oxygen or other oxidizing agents, leading to the formation of lithium oxide and antimony oxide.
Reduction: The compound can be reduced by strong reducing agents, although this is less common due to its already reduced state.
Intercalation: this compound is known for its ability to intercalate with other metals, such as silver and magnesium, forming compounds like Li₂AgSb and LiMgSb.
Major Products Formed:
Oxidation Products: Lithium oxide (Li₂O) and antimony oxide (Sb₂O₃).
Intercalation Products: Compounds such as Li₂AgSb and LiMgSb.
Aplicaciones Científicas De Investigación
Lithium antimonide has several scientific research applications, particularly in the field of energy storage. It is used as an anode material in lithium-ion and sodium-ion batteries due to its high theoretical capacity and suitable operating voltage . The compound’s unique puckered layer structure allows for high conductivity and reactivity with lithium and sodium ions, making it a promising candidate for high-performance batteries .
Its ability to form intermetallic compounds with other metals opens up possibilities for the development of new materials with enhanced properties .
Mecanismo De Acción
The mechanism of action of lithium antimonide in energy storage applications involves the intercalation and deintercalation of lithium ions. During the charging process, lithium ions are inserted into the antimonide structure, forming compounds such as Li₃Sb. This process is reversible, allowing the lithium ions to be extracted during discharge, thereby generating electrical energy .
At the molecular level, the intercalation of lithium ions leads to changes in the electronic and bonding properties of the antimonide. The covalent bonds in the parent phases of antimony are transformed into ionic bonds in the intercalated phases, enhancing the compound’s conductivity and stability .
Comparación Con Compuestos Similares
Copper Antimonide (Cu₂Sb)
Copper antimonide is a structurally stable intermetallic compound used in lithium-ion and sodium-ion battery anodes. Key distinctions from lithium antimonide include:
- Synthesis: Cu₂Sb is typically electrodeposited onto conductive substrates (e.g., copper foam or nanowires) .
- Applications : While SbLi₃ remains primarily experimental, Cu₂Sb has been commercialized in prototype batteries due to its scalable synthesis and compatibility with existing battery architectures .
Indium Antimonide (InSb)
A III-V semiconductor, InSb is distinct from this compound in electronic properties:
- Band Structure : InSb has a narrow bandgap (0.17 eV at 300 K), making it ideal for infrared photodetectors and high-speed transistors. In contrast, SbLi₃ is a metallic conductor .
- Crystal Structure : InSb adopts a zinc blende lattice, whereas SbLi₃’s structure is undefined in current literature .
- Applications : InSb is widely used in optoelectronics, while SbLi₃ is restricted to energy storage research .
Iron Antimonides (Hypothetical Fe-Sb Compounds)
Iron antimonides analogous to iron arsenide superconductors (e.g., LaFeAsO) remain hypothetical. Theoretical studies suggest Fe-Sb systems could exhibit superconductivity under high pressure, but synthesis challenges persist . In contrast, SbLi₃ is experimentally validated and actively researched for batteries .
Data Table: Key Properties of this compound and Comparable Compounds
Q & A
Basic Research Questions
Q. What experimental methods are recommended for synthesizing and characterizing Lithium Antimonide (Li₃Sb) crystals?
this compound synthesis typically involves solid-state reactions or electrodeposition. For high-purity crystals, use stoichiometric ratios of lithium and antimony in an inert atmosphere (e.g., argon) at elevated temperatures (~500–700°C) to minimize oxidation . Characterization requires X-ray diffraction (XRD) for crystal structure validation, scanning electron microscopy (SEM) for morphology, and energy-dispersive X-ray spectroscopy (EDS) for elemental composition. For electrochemical applications, cyclic voltammetry (CV) and galvanostatic cycling assess lithium-ion storage capacity .
Q. How can researchers determine the electronic band structure of this compound experimentally and computationally?
Experimental determination involves angle-resolved photoemission spectroscopy (ARPES) to map valence bands and optical absorption spectroscopy to identify direct/indirect bandgaps. Computational approaches like density functional theory (DFT) with hybrid functionals (e.g., HSE06) improve accuracy in predicting band parameters. Cross-validate results with magnetotransport measurements (e.g., Hall effect) to estimate carrier effective masses .
Q. What are the key electrochemical properties of this compound in lithium-ion battery anodes, and how are they optimized?
this compound exhibits high theoretical capacity (~660 mAh/g) due to its alloying mechanism with lithium. Optimize performance by synthesizing nanostructured forms (e.g., nanowires, nanoparticles) to reduce lithium diffusion paths and mitigate volume expansion. Use copper antimonide nanowires (1/50,000th human hair width) to enhance surface area and ion storage . Electrochemical impedance spectroscopy (EIS) identifies charge-transfer resistance, guiding electrolyte/electrode interface improvements .
Advanced Research Questions
Q. How does mechanical deformation influence the topological insulating properties of this compound?
Apply uniaxial stress or strain to single-crystal this compound and measure magnetoresistance at low temperatures (4.2–50 K) under high magnetic fields (0–14 T). Observe Shubnikov-de Haas (SdH) oscillations to detect Berry phase shifts (β ≈ 0.5), indicative of topological surface states. Compare with undeformed samples to isolate deformation-induced changes in carrier mobility and Fermi surface topology .
Q. What computational strategies resolve contradictions in reported carrier mobility values for this compound?
Discrepancies arise from defects (e.g., antisite defects, vacancies) and impurity scattering. Use DFT with Green’s function methods (e.g., DFT+Σ) to model defect formation energies and their impact on mobility. Experimentally, combine temperature-dependent Hall effect measurements with positron annihilation spectroscopy to quantify defect types and concentrations .
Q. How can researchers model the alloying/dealloying mechanisms of this compound during battery cycling?
Employ operando XRD or transmission electron microscopy (TEM) to track phase transitions (e.g., Li₃Sb ↔ Sb) in real time. Pair with finite-element simulations to predict stress distribution during lithiation. For kinetic analysis, use the GITT (galvanostatic intermittent titration technique) to extract diffusion coefficients and identify rate-limiting steps .
Q. Data Contradiction Analysis
Q. Why do studies report varying thermal stability thresholds for this compound in phase-change memory applications?
Differences arise from synthesis methods (e.g., vapor-liquid-solid vs. electrodeposition) affecting defect density. Thermal stability is assessed via differential scanning calorimetry (DSC) under controlled atmospheres. For phase-change studies, use in situ TEM to observe crystallization temperatures and validate against DFT-predicted activation energies .
Q. How to reconcile conflicting data on the role of antimony vacancies in this compound’s electronic properties?
Antimony vacancies act as acceptor defects, but their contribution depends on synthesis conditions. Use deep-level transient spectroscopy (DLTS) to characterize trap states. Cross-reference with photoluminescence (PL) spectra to distinguish vacancy-related transitions from other defects. Theoretical models must account for temperature-dependent vacancy migration .
Q. Methodological Tables
Table 1: Key Techniques for this compound Analysis
Table 2: Common Defects in this compound
Defect Type | Impact on Properties | Detection Method |
---|---|---|
Antisite (Li_Sb) | Reduces conductivity | EDS, DFT formation energy calculations |
Sb Vacancies | Increases hole concentration | PL, DLTS |
Grain Boundaries | Scatters carriers, lowers mobility | TEM, electron backscatter diffraction |
Propiedades
InChI |
InChI=1S/3Li.Sb/q3*+1; | |
---|---|---|
Source | PubChem | |
URL | https://pubchem.ncbi.nlm.nih.gov | |
Description | Data deposited in or computed by PubChem | |
InChI Key |
QCIJURNQAPERJR-UHFFFAOYSA-N | |
Source | PubChem | |
URL | https://pubchem.ncbi.nlm.nih.gov | |
Description | Data deposited in or computed by PubChem | |
Canonical SMILES |
[Li+].[Li+].[Li+].[Sb] | |
Source | PubChem | |
URL | https://pubchem.ncbi.nlm.nih.gov | |
Description | Data deposited in or computed by PubChem | |
Molecular Formula |
Li3Sb+3 | |
Source | PubChem | |
URL | https://pubchem.ncbi.nlm.nih.gov | |
Description | Data deposited in or computed by PubChem | |
Molecular Weight |
142.7 g/mol | |
Source | PubChem | |
URL | https://pubchem.ncbi.nlm.nih.gov | |
Description | Data deposited in or computed by PubChem | |
CAS No. |
12057-30-6 | |
Record name | Antimony, compd. with lithium (1:3) | |
Source | ChemIDplus | |
URL | https://pubchem.ncbi.nlm.nih.gov/substance/?source=chemidplus&sourceid=0012057306 | |
Description | ChemIDplus is a free, web search system that provides access to the structure and nomenclature authority files used for the identification of chemical substances cited in National Library of Medicine (NLM) databases, including the TOXNET system. | |
Record name | Antimony, compound with lithium (1:3) | |
Source | European Chemicals Agency (ECHA) | |
URL | https://echa.europa.eu/substance-information/-/substanceinfo/100.031.825 | |
Description | The European Chemicals Agency (ECHA) is an agency of the European Union which is the driving force among regulatory authorities in implementing the EU's groundbreaking chemicals legislation for the benefit of human health and the environment as well as for innovation and competitiveness. | |
Explanation | Use of the information, documents and data from the ECHA website is subject to the terms and conditions of this Legal Notice, and subject to other binding limitations provided for under applicable law, the information, documents and data made available on the ECHA website may be reproduced, distributed and/or used, totally or in part, for non-commercial purposes provided that ECHA is acknowledged as the source: "Source: European Chemicals Agency, http://echa.europa.eu/". Such acknowledgement must be included in each copy of the material. ECHA permits and encourages organisations and individuals to create links to the ECHA website under the following cumulative conditions: Links can only be made to webpages that provide a link to the Legal Notice page. | |
Descargo de responsabilidad e información sobre productos de investigación in vitro
Tenga en cuenta que todos los artículos e información de productos presentados en BenchChem están destinados únicamente con fines informativos. Los productos disponibles para la compra en BenchChem están diseñados específicamente para estudios in vitro, que se realizan fuera de organismos vivos. Los estudios in vitro, derivados del término latino "in vidrio", involucran experimentos realizados en entornos de laboratorio controlados utilizando células o tejidos. Es importante tener en cuenta que estos productos no se clasifican como medicamentos y no han recibido la aprobación de la FDA para la prevención, tratamiento o cura de ninguna condición médica, dolencia o enfermedad. Debemos enfatizar que cualquier forma de introducción corporal de estos productos en humanos o animales está estrictamente prohibida por ley. Es esencial adherirse a estas pautas para garantizar el cumplimiento de los estándares legales y éticos en la investigación y experimentación.