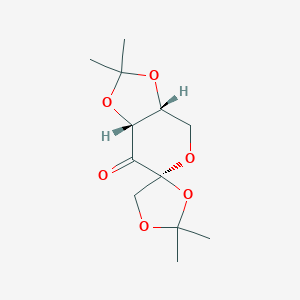
Shi Epoxidation Diketal Catalyst
Descripción general
Descripción
The Shi Epoxidation Diketal Catalyst (1,2:4,5-di-O-isopropylidene-β-D-erythro-2,3-hexodiulo-2,6-pyranose) is a chiral organocatalyst derived from D-fructose, renowned for enabling asymmetric epoxidation of alkenes without transition metals . Its mechanism involves the in situ generation of a chiral dioxirane intermediate via reaction with Oxone® (KHSO₅), which transfers oxygen to alkenes with high stereocontrol . Key features include:
- Substrate Scope: Effective for trans-1,2-disubstituted and trisubstituted alkenes, particularly styrene derivatives .
- Enantioselectivity: Achieves 90–97% enantiomeric excess (ee) for trans-alkenes, but struggles with cis-alkenes (e.g., 34% ee for cis-β-methylstyrene) .
- Reaction Conditions: Operates optimally at pH 10.5, enabling substoichiometric catalyst loadings (0.2–10 mol%) .
Mecanismo De Acción
Target of Action
The primary target of D-Epoxone, also known as the Shi Epoxidation Diketal Catalyst, is various alkenes . Alkenes are unsaturated hydrocarbons that contain at least one carbon-carbon double bond. They are a fundamental functional group in organic chemistry and serve as the building blocks for many complex molecules.
Mode of Action
D-Epoxone interacts with its target alkenes through a process known as epoxidation . This reaction involves the addition of an oxygen atom across the carbon-carbon double bond of the alkene, resulting in the formation of an epoxide . The epoxidizing species is believed to be a dioxirane , which is a powerful epoxidation reagent .
Biochemical Pathways
The Shi Epoxidation allows the synthesis of epoxides from various alkenes using a fructose-derived organocatalyst with Oxone as the primary oxidant . The reaction is believed to proceed via a dioxirane intermediate generated from the ketone catalyst by the Oxone . The downstream effects of this reaction include the creation of a variety of complex molecules, as epoxides are versatile intermediates in organic synthesis.
Pharmacokinetics
It’s known that d-epoxone is a chemical reagent used for enantioselective epoxidation of alkenes . It’s involved in the synthesis of Irciniastatin (A and B), which are used as antitumor compounds . It’s used as a cytotoxic secondary metabolite .
Result of Action
The result of D-Epoxone’s action is the formation of epoxides from various alkenes . Epoxides are cyclic ethers that are important in a variety of chemical reactions, including those in biological systems. They can be opened by nucleophiles to form a range of products, making them valuable intermediates in organic synthesis.
Action Environment
The Shi Epoxidation reaction is sensitive to environmental factors such as pH and temperature . The optimum pH for dioxirane epoxidation is 7-8 . At higher pH, Oxone tends to decompose . At ph 7-8 the shi catalyst decomposes due to competing baeyer-villiger reaction . By increasing the pH to 10.5 (by addition of K2CO3), the amount of ketone used can be reduced to a catalytic amount (30 mol %) and the amount of Oxone can be reduced to a stoichiometric amount (1.5 equiv), suggesting that at this pH the ketone is sufficiently reactive to compete with Oxone decomposition . Reaction temperatures range from –10 to 20 °C .
Actividad Biológica
The Shi epoxidation diketal catalyst is a significant compound in the realm of asymmetric epoxidation, particularly due to its unique biological activity and catalytic properties. This article explores its biological activity, mechanisms, and applications, supported by data tables and case studies.
Overview of this compound
The this compound is primarily utilized for the asymmetric epoxidation of alkenes. It is known for its ability to produce enantiomerically enriched epoxides, which are crucial intermediates in the synthesis of various pharmaceuticals and agrochemicals. The catalyst operates through a mechanism involving the formation of reactive intermediates that facilitate the selective oxidation of alkenes.
The mechanism of action for the this compound involves the following steps:
- Formation of the Active Species : The diketal undergoes auto-oxidation to form an active oxo-species.
- Epoxidation Reaction : This active species reacts with alkenes to form epoxides with high enantioselectivity.
- Regeneration : The catalyst can be regenerated under specific conditions, maintaining its effectiveness over multiple cycles.
Enantioselectivity and Reaction Conditions
Research indicates that the this compound exhibits high enantioselectivity in the production of epoxides. For instance, in a study involving styrene monooxygenase from Rhodococcus sp., it was found that the Shi catalyst produced the opposite enantiomer with an enantiomeric excess (ee) exceeding 90% under optimized conditions .
Table 1: Enantiomeric Excess of Epoxides Produced by Different Catalysts
Catalyst | Substrate | Enantiomeric Excess (%) |
---|---|---|
Shi Epoxidation Diketal | Styrene | >90 |
Jacobsen Catalyst (R,R) | Styrene | >95 |
Jacobsen Catalyst (S,S) | Styrene | >95 |
Case Study 1: Asymmetric Epoxidation of Alkenes
In a detailed investigation, the this compound was employed to convert various alkenes into their corresponding epoxides. The study highlighted that using acetonitrile as a solvent significantly influenced both conversion rates and enantiomeric purity. When acetonitrile concentration was reduced, a notable decrease in conversion efficiency was observed, indicating its critical role in the reaction mechanism .
Table 2: Influence of Solvent Concentration on Epoxide Yield
Acetonitrile Concentration (%) | Conversion Rate (%) | Enantiomeric Excess (%) |
---|---|---|
10 | 85 | 80-85 |
5 | 50 | 50 |
Case Study 2: Cascade Reactions
A recent study explored one-pot two-step cascade reactions using the this compound followed by an enzyme-catalyzed ring-opening reaction. The results demonstrated that while the Shi catalyst effectively produced epoxides, subsequent steps were sensitive to reaction conditions such as pH and temperature. The integration of whole-cell biocatalysts improved overall yield but required careful optimization to prevent degradation of intermediates .
Research Findings
The biological activity of the this compound has been extensively documented through various studies:
- Catalytic Efficiency : The catalyst shows remarkable efficiency in converting alkenes to epoxides with high selectivity.
- Compatibility with Biocatalysts : When combined with biocatalysts such as hydroxylated dehydrogenases (HHDHs), it enhances overall reaction efficiency but requires specific conditions for optimal performance .
- Environmental Impact : The use of this catalyst aligns with sustainable chemistry practices by enabling reactions under mild conditions and minimizing waste.
Análisis De Reacciones Químicas
Core Reaction Mechanism
The catalyst operates via a dioxirane intermediate generated in situ by oxidizing the ketone group with Oxone (potassium peroxymonosulfate). Key steps include:
-
Catalyst Activation : Oxone oxidizes the ketone to form a highly reactive dioxirane species .
-
Epoxidation : The dioxirane transfers an oxygen atom to the alkene, forming an epoxide with stereochemical control from the catalyst’s chiral centers2 .
-
Catalyst Regeneration : The ketone is regenerated, enabling catalytic turnover .
The rigid bicyclic structure of the catalyst minimizes epimerization, ensuring high enantioselectivity .
Substrate Scope and Selectivity
The catalyst exhibits broad applicability for trans-alkenes and select cis-alkenes , with enantiomeric excess (ee) often exceeding 90% . Notable examples:
Substrate Type | ee (%) | Yield (%) | Conditions | Source |
---|---|---|---|---|
Styrene derivatives | >90 | 85–95 | Oxone, CH₃CN, K₂CO₃ | |
Disulfides | 96 | 89 | Oxone, CH₃CN/Dimethoxymethane | |
Trisubstituted alkenes | 88 | 78 | Oxone, buffered pH 10.5 |
Limitations : Electron-deficient alkenes and highly strained systems show reduced reactivity .
Solvent Effects
Acetonitrile (CH₃CN) is pivotal for maintaining high conversion and enantioselectivity:
CH₃CN Concentration (%) | Conversion (%) | ee (%) |
---|---|---|
10 | 85 | 80–85 |
5 | 50 | 50 |
Lower CH₃CN levels promote non-selective auto-oxidation of the catalyst, reducing efficiency .
pH and Temperature
-
Optimal pH : 10.5 (buffered with K₂CO₃) minimizes side reactions like Baeyer-Villiger oxidation .
-
Temperature : Reactions typically proceed at 0–25°C; higher temperatures reduce enantioselectivity .
Pharmaceutical Intermediates
-
Sphingosine Analogues : Shi epoxidation enabled a key step in synthesizing 2-amino-3,5-diols, potential anticancer agents .
-
Chiral Thiosulfonates : Achieved 96% ee in sulfinyl derivatives, used as chiral ligands .
Cascade Reactions
-
Enzyme-Compatible Systems : Combined Shi epoxidation with enzymatic ring-opening reactions in one-pot cascades, though sensitivity to pH and temperature required optimization .
Comparison with Other Epoxidation Methods
Catalyst | Substrate Preference | ee (%) | Oxidant | Metal-Free |
---|---|---|---|---|
Shi Diketal Catalyst | trans-alkenes | 90–96 | Oxone/H₂O₂ | Yes |
Jacobsen (Mn-salen) | cis-alkenes | 95 | NaOCl | No |
Sharpless | Allylic alcohols | 90 | t-BuOOH | No |
Advantages : Metal-free, scalable, and compatible with green chemistry principles .
Synthetic Modifications
Recent advancements include using hydrogen peroxide instead of Oxone, reducing salt byproducts and solvent waste .
Q & A
Basic Research Questions
Q. What is the mechanistic role of pH in Shi epoxidation reactions, and how can it be optimized for enantioselectivity?
The Shi catalyst's activity is highly pH-dependent. At pH >10 (achieved via K₂CO₃ addition), the reaction rate increases tenfold while maintaining high enantioselectivity (90–92%) for trans-disubstituted and trisubstituted olefins. This is attributed to enhanced dioxirane intermediate formation under basic conditions . Control experiments at lower pH (7–8) show minimal conversion (3.3%) and selectivity (17.7%) due to incomplete activation of the ketone catalyst .
Q. How should experimental controls be designed to validate the catalytic role of the Shi diketal catalyst in epoxidation?
Key controls include:
- Blank reactions : Exclude the catalyst to confirm its necessity.
- Oxidant-free tests : Omit Oxone® to verify its role in dioxirane generation.
- Substrate scope analysis : Test trans- vs. cis-olefins to assess stereochemical limitations (e.g., cis-olefins often yield lower ee%) . For example, in soybean oil epoxidation, removing the oxidant (TBHP) reduced conversion to 3.3%, highlighting its critical role in oxygen transfer .
Q. What are the substrate limitations of the Shi catalyst, and how can they be addressed methodologically?
The Shi catalyst excels in epoxidizing trans-disubstituted and trisubstituted alkenes (ee >90%) but struggles with terminal and cis-olefins. To mitigate this:
- Use co-solvent systems (e.g., CH₃CN/H₂O) to improve solubility and catalyst-substrate interactions.
- Optimize steric and electronic effects via substrate derivatization (e.g., introducing electron-withdrawing groups) .
- Consider hybrid catalysts (e.g., immobilized organocatalysts) for enhanced stereocontrol in challenging cases .
Advanced Research Questions
Q. How can computational modeling (e.g., DFT) resolve contradictions in experimental enantioselectivity data?
DFT studies reveal that enantioselectivity arises from noncovalent interactions (e.g., hydrogen bonding) between the substrate and the oxazolidinone moiety of the catalyst. For example, in cis-enyne epoxidation, steric clashes between the alkyne and catalyst reduce ee%, which aligns with experimental observations of <50% ee for such substrates . Microkinetic models integrating these interactions can predict catalytic outcomes for novel substrates .
Q. What factors contribute to catalyst deactivation, and how can stability be improved?
Deactivation mechanisms include:
- Oxidative degradation : Prolonged exposure to Oxone® degrades the ketone catalyst.
- Aggregation : Heterogeneous conditions (e.g., in nonpolar solvents) reduce active site accessibility . Solutions:
- Heterogenization : Immobilize the catalyst on nanoporous silica to enhance recyclability .
- Additives : Introduce stabilizing agents (e.g., polyvinylpyrrolidone) to prevent aggregation .
Q. How can advanced characterization techniques (e.g., in situ spectroscopy) elucidate the Shi catalyst's active species?
- Raman spectroscopy : Identifies the dioxirane intermediate (ν(O–O) ~550 cm⁻¹) during Oxone® activation .
- XAS/XPS : Tracks electronic state changes in the ketone catalyst under reaction conditions .
- TEM/STEM : Monitors morphological stability in heterogenized catalysts .
Q. What methodologies reconcile contradictory data on solvent effects in Shi epoxidation?
Contradictions arise from solvent polarity impacts on transition-state stabilization. For example:
- Polar aprotic solvents (e.g., CH₃CN) enhance ee% by stabilizing hydrogen-bonded intermediates.
- Nonpolar solvents (e.g., toluene) reduce conversion due to poor catalyst solubility . Systematic solvent screening via Design of Experiments (DoE) can optimize polarity and proticity .
Q. Methodological Guidelines
Q. How to ensure reproducibility in Shi epoxidation protocols?
- Standardize oxidant purity : Use fresh Oxone® (≥47% KHSO₅) to avoid batch variability.
- Control moisture : Strict anhydrous conditions prevent catalyst hydrolysis.
- Document pH precisely : Use calibrated probes to maintain pH 10.5 (±0.1) .
Q. What statistical approaches are recommended for optimizing reaction conditions?
Comparación Con Compuestos Similares
Comparison with Similar Catalysts
Transition Metal-Based Catalysts
Jacobsen’s Mn(III)-Salen Catalyst
- Mechanism : Utilizes Mn(III)-salen complexes for epoxidation, requiring stoichiometric oxidants like NaOCl .
- Performance :
- Drawbacks : Transition metal residues complicate purification and raise environmental concerns .
Table 1: Shi vs. Jacobsen Catalysts
Vanadium Schiff Base Complexes
- Mechanism : Vanadium centers activate peroxides for epoxidation .
- Performance :
- Drawbacks : Requires transition metals and often harsher conditions .
Enzymatic Catalysts
Styrene Monooxygenase (StyAB)
- Mechanism : Enzymatic epoxidation using molecular oxygen and NADH .
- Performance: Enantioselectivity: >95% ee for (S,S)-epoxides . Substrate Compatibility: Limited to terminal alkenes (e.g., styrene derivatives).
- Drawbacks : Low substrate tolerance (<20 mM) and challenges in cofactor regeneration .
Table 2: Shi vs. StyAB in Cascade Reactions
Parameter | Shi Catalyst + HHDH | StyAB + HHDH |
---|---|---|
Epoxide ee | ~60% | >99% |
Conversion | 30–36% | 20–39% |
Regioselectivity | Moderate | High |
Solvent Compatibility | Requires acetonitrile (10%) | Aqueous buffer |
Key Reference |
Heterogeneous Catalysts
Au/Ti-MWW Zeolite
- Mechanism: Au nanoparticles on Ti-MWW activate H₂ and O₂ for propylene epoxidation .
- Performance :
Metal-Organic Frameworks (MOFs)
- Example : UiO-66-NH₂ immobilized Mn(III)-salen .
- Requires tert-butyl hydroperoxide (TBHP) as an oxidant .
Key Research Findings and Challenges
Substrate Limitations :
- Shi catalyst underperforms with cis-alkenes and electron-deficient alkenes .
- Enzymatic catalysts (e.g., StyAB) face substrate concentration limits (<50 mM) .
Solvent and Compatibility Issues :
- Shi-based cascades require acetonitrile, which inhibits downstream enzymes (e.g., HHDH) .
- Heterogeneous catalysts (e.g., MOFs) suffer from pore diffusion limitations .
Environmental and Practical Considerations :
Métodos De Preparación
Synthetic Pathways and Key Reaction Steps
Core Synthesis from D-Fructose
The catalyst is synthesized through a multi-step sequence starting with D-fructose , which undergoes successive protection and oxidation reactions:
-
Ketal Formation :
-
Selective Oxidation :
Table 1: Comparison of Oxidation Methods
Method | Oxidant | Temperature (°C) | Diketone Purity (%) | Environmental Impact |
---|---|---|---|---|
Jones Reagent | CrO₃/H₂SO₄ | 0–5 | 88–92 | High (Cr waste) |
TEMPO/NaClO | NaClO, NaBr | 25 | 94–96 | Moderate |
Pt/C + O₂ | Molecular oxygen | 50 | 90–93 | Low |
Industrial-Scale Production Challenges
Solvent System Optimization
Large-scale synthesis requires balancing reaction efficiency with solvent recovery:
-
Preferred solvent : Anhydrous acetone (recyclability ≥85%)
-
Alternative systems:
Crystallization and Purity Control
Final product isolation involves:
-
Anti-solvent precipitation : Adding cold hexane to reaction mixtures induces crystallization.
-
Chromatographic polishing : Required for pharmaceutical-grade material (≥99.5% purity).
Critical quality attributes :
-
Residual acetone: ≤0.5% (GC analysis)
-
Isopropylidene group integrity: Confirmed via ¹³C NMR (δ 109–112 ppm for ketal carbons) .
Recent Methodological Innovations
Continuous Flow Synthesis
Pilot-scale studies demonstrate advantages over batch processes:
-
Microreactor system :
Biocatalytic Approaches
Emerging hybrid strategies combine chemical and enzymatic steps:
-
Lipase-mediated protection :
-
Limitations:
Parameter | Specification | Rationale |
---|---|---|
Temperature | 2–8°C | Minimizes thermal degradation |
Humidity | ≤30% RH (desiccator) | Prevents hydrolysis |
Light | Amber glass containers | Reduces photo-oxidation |
Shelf life | 24 months (unopened) | Validated by accelerated testing |
Analytical Characterization Standards
Spectroscopic Benchmarks
¹H NMR (400 MHz, CDCl₃) :
FT-IR (KBr) :
Chromatographic Purity Methods
Technique | Column | Mobile Phase | Retention Time (min) |
---|---|---|---|
HPLC-UV (210 nm) | Zorbax SB-C18 (4.6×150 mm) | Acetonitrile/water (70:30) | 6.8 ± 0.2 |
GC-FID | HP-5MS (30 m × 0.25 mm) | Helium, 1.5 mL/min | 12.4 |
Industrial Case Studies
Pharmaceutical Intermediate Production
A 2024 study detailed catalyst performance in synthesizing antiviral drug intermediates :
-
Scale : 200 kg batch
-
Key metrics :
Agrochemical Applications
In herbicide precursor synthesis:
-
Process modification :
Component | OSHA PEL (8-h TWA) | NIOSH REL |
---|---|---|
Acetonitrile | 40 ppm | 20 ppm |
TEMPO | Not established | 0.1 mg/m³ (proposed) |
Propiedades
IUPAC Name |
(3'aR,4S,7'aR)-2,2,2',2'-tetramethylspiro[1,3-dioxolane-4,6'-4,7a-dihydro-3aH-[1,3]dioxolo[4,5-c]pyran]-7'-one | |
---|---|---|
Source | PubChem | |
URL | https://pubchem.ncbi.nlm.nih.gov | |
Description | Data deposited in or computed by PubChem | |
InChI |
InChI=1S/C12H18O6/c1-10(2)15-6-12(18-10)9(13)8-7(5-14-12)16-11(3,4)17-8/h7-8H,5-6H2,1-4H3/t7-,8-,12+/m1/s1 | |
Source | PubChem | |
URL | https://pubchem.ncbi.nlm.nih.gov | |
Description | Data deposited in or computed by PubChem | |
InChI Key |
IVWWFWFVSWOTLP-RWYTXXIDSA-N | |
Source | PubChem | |
URL | https://pubchem.ncbi.nlm.nih.gov | |
Description | Data deposited in or computed by PubChem | |
Canonical SMILES |
CC1(OCC2(O1)C(=O)C3C(CO2)OC(O3)(C)C)C | |
Source | PubChem | |
URL | https://pubchem.ncbi.nlm.nih.gov | |
Description | Data deposited in or computed by PubChem | |
Isomeric SMILES |
CC1(OC[C@]2(O1)C(=O)[C@H]3[C@@H](CO2)OC(O3)(C)C)C | |
Source | PubChem | |
URL | https://pubchem.ncbi.nlm.nih.gov | |
Description | Data deposited in or computed by PubChem | |
Molecular Formula |
C12H18O6 | |
Source | PubChem | |
URL | https://pubchem.ncbi.nlm.nih.gov | |
Description | Data deposited in or computed by PubChem | |
DSSTOX Substance ID |
DTXSID70428499 | |
Record name | D-Epoxone | |
Source | EPA DSSTox | |
URL | https://comptox.epa.gov/dashboard/DTXSID70428499 | |
Description | DSSTox provides a high quality public chemistry resource for supporting improved predictive toxicology. | |
Molecular Weight |
258.27 g/mol | |
Source | PubChem | |
URL | https://pubchem.ncbi.nlm.nih.gov | |
Description | Data deposited in or computed by PubChem | |
CAS No. |
18422-53-2 | |
Record name | D-Epoxone | |
Source | EPA DSSTox | |
URL | https://comptox.epa.gov/dashboard/DTXSID70428499 | |
Description | DSSTox provides a high quality public chemistry resource for supporting improved predictive toxicology. | |
Descargo de responsabilidad e información sobre productos de investigación in vitro
Tenga en cuenta que todos los artículos e información de productos presentados en BenchChem están destinados únicamente con fines informativos. Los productos disponibles para la compra en BenchChem están diseñados específicamente para estudios in vitro, que se realizan fuera de organismos vivos. Los estudios in vitro, derivados del término latino "in vidrio", involucran experimentos realizados en entornos de laboratorio controlados utilizando células o tejidos. Es importante tener en cuenta que estos productos no se clasifican como medicamentos y no han recibido la aprobación de la FDA para la prevención, tratamiento o cura de ninguna condición médica, dolencia o enfermedad. Debemos enfatizar que cualquier forma de introducción corporal de estos productos en humanos o animales está estrictamente prohibida por ley. Es esencial adherirse a estas pautas para garantizar el cumplimiento de los estándares legales y éticos en la investigación y experimentación.