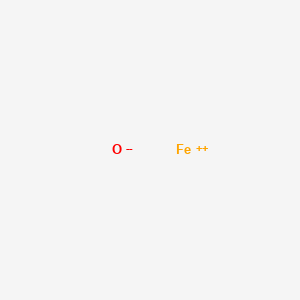
Iron oxide
Übersicht
Beschreibung
Iron oxides are a class of chemical compounds composed of iron and oxygen, with diverse structures and oxidation states. The most common forms include hematite (α-Fe₂O₃), magnetite (Fe₃O₄), and maghemite (γ-Fe₂O₃). These compounds exhibit unique physicochemical properties such as magnetism, catalytic activity, and biocompatibility, making them indispensable in fields ranging from environmental remediation to biomedicine and electronics .
Key properties include:
- Magnetic behavior: Magnetite and maghemite are ferrimagnetic, while hematite is weakly magnetic.
- Chemical stability: Hematite and maghemite are highly stable under ambient conditions, whereas magnetite oxidizes to maghemite in air .
- Catalytic activity: Iron oxides participate in redox reactions, enabling applications in Fenton systems and peroxidase-like catalysis .
Vorbereitungsmethoden
Chemical Coprecipitation: The Foundation of Iron Oxide Synthesis
Chemical coprecipitation remains the most widely adopted method for this compound nanoparticle synthesis due to its simplicity, scalability, and cost-effectiveness. This technique involves the simultaneous precipitation of Fe²⁺ and Fe³⁺ ions in an aqueous solution under alkaline conditions. The molar ratio of Fe²⁺ to Fe³⁺ is critical, with a 1:2 ratio being optimal for magnetite formation . The general reaction proceeds as follows:
3\text{O}4 + 4\text{H}_2\text{O}
Key parameters influencing particle characteristics include pH, temperature, ionic strength, and the choice of base (e.g., NaOH, NH₄OH) . For instance, higher pH values (>10) favor magnetite formation, while lower pH values (7–9) may yield goethite (α-FeOOH) or lepidocrocite (γ-FeOOH) . Particle size can be modulated by adjusting mixing rates or introducing surfactants such as isobutanol, which acts as a stabilizing agent to prevent agglomeration .
Phase Transformation Through Calcination
Post-synthesis calcination enables phase control. Magnetite (Fe₃O₄) converts to maghemite (γ-Fe₂O₃) at 300°C in air, while temperatures exceeding 500°C yield hematite (α-Fe₂O₃) . For example, calcining Fe₃O₄ at 530°C for 2 hours produces α-Fe₂O₃ nanoparticles with a rhombohedral morphology .
Table 1: Impact of Calcination Conditions on this compound Phase
Precursor | Temperature (°C) | Time (h) | Phase Obtained | Particle Size (nm) |
---|---|---|---|---|
Fe₃O₄ | 300 | 1.7 | γ-Fe₂O₃ | 11–22 |
Fe₃O₄ | 530 | 2 | α-Fe₂O₃ | 22–45 |
FeOOH | 600 | 5 | α-Fe₂O₃ | 50–100 |
Electrochemical Synthesis: Precision Through Controlled Redox Reactions
Electrochemical methods offer enhanced control over particle size and crystallinity by leveraging redox reactions at electrodes. In a typical setup, an iron anode is oxidized to release Fe²⁺ ions, while water reduction at the cathode generates OH⁻ ions :
Anode (Oxidation):
Cathode (Reduction):
2\text{O} + 2\text{e}^- \rightarrow \text{H}2 + 2\text{OH}^-
The Fe²⁺ and OH⁻ ions react to form Fe(OH)₂, which further oxidizes to Fe₃O₄ in the presence of dissolved oxygen . This method avoids the rapid precipitation seen in coprecipitation, enabling finer control over nucleation and growth. For instance, Aghazadeh et al. (2017) demonstrated that adjusting the current density from 10 to 50 mA/cm² reduces particle size from 45 nm to 12 nm .
Hydrothermal Synthesis: High-Pressure Crystallization
Hydrothermal synthesis involves reacting iron precursors (e.g., FeCl₃, FeSO₄) in aqueous solutions at elevated temperatures (120–250°C) and pressures (1–10 MPa). This method facilitates the growth of well-crystallized particles with minimal defects. For example, reacting FeCl₃ and urea at 180°C for 12 hours produces α-Fe₂O₃ nanorods with diameters of 30–50 nm . The alkaline environment and prolonged reaction time favor Ostwald ripening, leading to uniform morphology.
Table 2: Hydrothermal Synthesis Parameters and Outcomes
Precursor | Temperature (°C) | Time (h) | Additive | Phase | Morphology |
---|---|---|---|---|---|
FeCl₃ + urea | 180 | 12 | None | α-Fe₂O₃ | Nanorods |
FeSO₄ + NaOH | 200 | 24 | CTAB | γ-Fe₂O₃ | Spheres |
Fe(NO₃)₃ | 150 | 6 | Oleic acid | Fe₃O₄ | Octahedrons |
Sol-Gel Synthesis: Tailoring Morphology Through Precursor Chemistry
The sol-gel method involves hydrolyzing iron alkoxides (e.g., Fe(OCH₃)₃) to form a colloidal sol, which is subsequently gelled and calcined. Acid or base catalysts influence the gelation kinetics and final structure. For instance, using nitric acid as a catalyst promotes the formation of mesoporous γ-Fe₂O₃ with a surface area of 150 m²/g . This method is particularly effective for producing thin films or composites, as the sol can be spin-coated or dip-coated onto substrates.
Thermal Decomposition: High-Temperature Phase Control
Thermal decomposition of iron-containing precursors (e.g., iron oxalate, iron acetylacetonate) in inert or reducing atmospheres enables precise phase control. For example, decomposing FeC₂O₄·2H₂O at 400°C under N₂ yields Fe₃O₄, while decomposition in air produces α-Fe₂O₃ . Particle size is influenced by heating rate; slower rates (2°C/min) yield larger crystals (80 nm) compared to faster rates (10°C/min, 20 nm) .
Critical Factors Influencing this compound Synthesis
pH and Oxidation Rate
The solution pH dictates the solubility of iron species and the nucleation rate. At pH < 4, Fe³⁺ remains soluble, whereas pH > 9 promotes rapid precipitation of Fe₃O₄ . Oxidation rate also affects phase composition: slow oxidation (5% O₂) favors α-FeOOH, while rapid oxidation (100% O₂) yields γ-FeOOH .
Temperature and Ionic Strength
Higher temperatures (e.g., 308 K) accelerate particle growth, increasing crystallinity but also promoting agglomeration . Ionic strength modulates electrostatic interactions between particles; for example, Na⁺ ions compress the double layer, reducing repulsion and increasing aggregation .
Analyse Chemischer Reaktionen
Formation of Iron Oxides
Iron reacts with oxygen under high-temperature conditions to form three primary oxides:
These reactions occur during combustion, with Fe₃O₄ ("iron cinder") forming as a mixed iron oxide when iron burns at high temperatures .
Acidic Conditions
Iron oxides react with strong acids like sulfuric acid:
In dilute sulfuric acid, Fe dissolves to form Fe²⁺ ions and hydrogen gas:
Basic Conditions
Iron oxides react with hydroxide ions to form hydroxides:
Heating these hydroxides dehydrates them back to oxides .
Environmental Interactions
Iron oxides participate in redox cycles and environmental processes:
Thermochemical Data for FeO
Property | Value | Units | Source |
---|---|---|---|
Standard molar enthalpy of formation (ΔfH°) | -272.04 | kJ/mol | |
Standard molar entropy (S°) | 60.75 | J/mol·K |
Rusting Process
Iron metal reacts with moist air to form hydrated this compound (Fe(OH)₃), which flakes off, exposing fresh metal for further oxidation . This process is critical in corrosion science.
Advanced Oxide-Hydroxides
This compound-hydroxides like goethite (α-FeOOH) and ferrihydrite exhibit unique reactivity:
-
Catalytic decomposition of H₂O₂ : α-FeOOH accelerates H₂O₂ breakdown via surface-mediated reactions .
-
Cluster reactivity : FeO₂ and FeO₃ clusters react with CO, while larger clusters (e.g., Fe₂O₄) are inert .
Thermochemical Cycles
The This compound cycle enables hydrogen production via redox steps:
Reduction :
Oxidation :
This cycle operates at high temperatures (1200–1400°C) and utilizes solar/geothermal energy .
Wissenschaftliche Forschungsanwendungen
Ferrous oxide has numerous applications in scientific research, including:
Wirkmechanismus
Ferrous oxide exerts its effects through various mechanisms, including:
Catalysis: Acts as a catalyst in chemical reactions by providing active sites for reactants to interact.
Magnetic Properties: Its magnetic properties are exploited in applications such as magnetic storage and separation.
Biological Interactions: In biological systems, ferrous oxide can participate in redox reactions, influencing cellular processes and iron metabolism.
Vergleich Mit ähnlichen Verbindungen
Iron Oxides vs. Ferrous Sulfate (FeSO₄)
Iron oxide nanoparticles (e.g., Fe₃O₄) and ferrous sulfate are both used as iron supplements. A 1996 study comparing their bioavailability in rabbits found:
Parameter | This compound (Fe₃O₄) | Ferrous Sulfate (FeSO₄) |
---|---|---|
Serum Iron Increase | Comparable | Slightly Higher |
Absorption Efficiency | 85–90% | 90–95% |
Toxicity | Lower | Higher (GI irritation) |
This compound’s slower release and reduced toxicity make it favorable for long-term supplementation .
Magnetite (Fe₃O₄) vs. Maghemite (γ-Fe₂O₃)
These two spinel-structured oxides are often compared due to their magnetic properties:
Property | Magnetite (Fe₃O₄) | Maghemite (γ-Fe₂O₃) |
---|---|---|
Oxidation State | Mixed Fe²⁺/Fe³⁺ | Fe³⁺ only |
Magnetic Saturation | 92 emu/g | 76 emu/g |
Stability | Oxidizes in air | Air-stable |
Catalytic Role in Fenton Systems | More active due to Fe²⁺ | Less active |
Magnetite’s Fe²⁺ content enhances its peroxidase-like activity and utility in wastewater treatment, while maghemite’s stability favors biomedical imaging .
Hematite (α-Fe₂O₃) vs. Lepidocrocite (γ-FeOOH)
Hematite and lepidocrocite differ in structure and reactivity:
Property | Hematite (α-Fe₂O₃) | Lepidocrocite (γ-FeOOH) |
---|---|---|
Crystal Structure | Hexagonal | Orthorhombic |
Bandgap | 2.2 eV | 2.6 eV |
Photocatalytic Activity | Moderate | High (due to layered structure) |
Environmental Role | Adsorbs heavy metals | Degrades organic pollutants |
Hematite is widely used in pigments and sensors, while lepidocrocite’s layered structure enhances catalytic degradation of organics .
Q & A
Basic Research Questions
Q. How can researchers determine the empirical formula of an iron oxide compound using mass composition data?
Methodological Answer: To determine the empirical formula, conduct stoichiometric calculations based on mass percentages of Fe and O. For example, if an oxide contains 69.9% Fe and 30.1% O by mass:
- Convert mass to moles: Fe = 69.9/55.85 ≈ 1.25 mol; O = 30.1/16 ≈ 1.88 mol.
- Determine the simplest ratio: Fe:O ≈ 1:1.5 → Multiply by 2 to eliminate decimals → Fe₂O₃. Validate using spectroscopic techniques (e.g., XRD for crystallography or XPS for oxidation states) .
Q. What are the standard laboratory methods for synthesizing this compound nanoparticles, and how do they influence particle properties?
Methodological Answer: Common methods include:
- Co-precipitation : Adjust pH of Fe²⁺/Fe³⁺ solutions with a base (e.g., NH₄OH). Controls size via reaction kinetics .
- Hydrothermal synthesis : Uses high-temperature autoclaves to produce crystalline nanoparticles (e.g., α-Fe₂O₃). Particle morphology depends on temperature and precursor concentration .
- Sol-gel : Hydrolysis of iron alkoxides yields high-purity oxides but requires careful drying to prevent aggregation. Characterize outcomes using TEM (size), XRD (crystallinity), and VSM (magnetic properties) .
Advanced Research Questions
Q. How can researchers optimize the photocatalytic degradation of organic pollutants using this compound nanoparticles?
Methodological Answer: Use Response Surface Methodology (RSM) with a Central Composite Design (CCD) to model interactions between variables:
- Parameters: Catalyst loading (5–20 mg/L), pH (3–9), pollutant concentration (10–50 ppm), and irradiation time (30–120 min).
- Analyze variance (ANOVA) to identify significant factors. For example, pH 5–7 enhances Fe₃O₄ activity due to optimal surface charge . Validate with UV-Vis spectroscopy to track pollutant degradation kinetics .
Q. What experimental strategies address contradictions in adsorption efficiency data for this compound-based nanocomposites?
Methodological Answer:
- Standardize synthesis : Use identical precursors (e.g., FeCl₃·6H₂O and FeCl₂·4H₂O for magnetite) and reaction conditions (e.g., N₂ atmosphere to prevent oxidation) .
- Control characterization : Compare BET surface area, pore size, and zeta potential across studies. For instance, Fe₃O₄/Bentonite nanocomposites show higher 4-nitrophenol adsorption (98%) at pH 6 due to electrostatic interactions .
- Statistical reconciliation : Apply multivariate regression to isolate variables (e.g., adsorbent dosage vs. contact time) .
Q. How can in vivo studies assess the biocompatibility of this compound nanoparticles in aquatic organisms?
Methodological Answer:
- Experimental design : Expose model organisms (e.g., Labeo rohita) to nanoparticles (10–100 mg/kg diet) over 60 days. Monitor growth metrics (weight gain, feed conversion ratio) and hematological parameters (hemoglobin, RBC count) .
- Tissue analysis : Use ICP-MS to quantify iron accumulation in muscle and liver. Histopathology can reveal oxidative stress markers (e.g., lipid peroxidation) .
- Dose-response modeling : Fit data to logarithmic curves to determine NOAEL (No Observed Adverse Effect Level) .
Q. What methodologies resolve inconsistencies in the catalytic activity of iron oxides across redox reactions?
Methodological Answer:
- Surface modification : Functionalize Fe₂O₃ with SiO₂ or polymers to stabilize active sites. For example, SiO₂-coated γ-Fe₂O₃ shows 30% higher CO oxidation efficiency at 300°C .
- Operando characterization: Use XAFS or FTIR during reactions to track surface intermediates and oxidation state changes (e.g., Fe³⁺ ↔ Fe²⁺) .
- Controlled atmosphere studies : Conduct experiments under inert (N₂) or reactive (O₂) gases to isolate redox mechanisms .
Q. Methodological Challenges and Solutions
Q. How should researchers design experiments to account for phase transformations in iron (hydr)oxides during synthesis?
Methodological Answer:
- In situ monitoring : Use XRD or Raman spectroscopy during synthesis to detect phase changes (e.g., lepidocrocite → magnetite) .
- Post-synthesis stabilization : Anneal samples at 400°C to convert amorphous phases to crystalline α-Fe₂O₃ .
- Document pretreatment steps : Report drying temperatures and storage conditions to ensure reproducibility .
Q. What statistical approaches are recommended for analyzing heterogeneous datasets in this compound research?
Methodological Answer:
- Multivariate analysis : Use PCA (Principal Component Analysis) to reduce dimensionality in datasets with >10 variables (e.g., synthesis parameters, adsorption efficiency) .
- Meta-analysis : Aggregate data from 15+ studies to identify trends. For example, Fe₃O₄ nanoparticles exhibit a median adsorption capacity of 120 mg/g for heavy metals, with 95% confidence intervals .
- Machine learning : Train neural networks on historical data to predict optimal synthesis conditions (e.g., 85% accuracy for particle size <50 nm) .
Eigenschaften
Molekularformel |
FeO |
---|---|
Molekulargewicht |
71.84 g/mol |
IUPAC-Name |
iron(2+);oxygen(2-) |
InChI |
InChI=1S/Fe.O/q+2;-2 |
InChI-Schlüssel |
VBMVTYDPPZVILR-UHFFFAOYSA-N |
Kanonische SMILES |
[O-2].[Fe+2] |
Herkunft des Produkts |
United States |
Synthesis routes and methods I
Procedure details
Synthesis routes and methods II
Procedure details
Synthesis routes and methods III
Procedure details
Haftungsausschluss und Informationen zu In-Vitro-Forschungsprodukten
Bitte beachten Sie, dass alle Artikel und Produktinformationen, die auf BenchChem präsentiert werden, ausschließlich zu Informationszwecken bestimmt sind. Die auf BenchChem zum Kauf angebotenen Produkte sind speziell für In-vitro-Studien konzipiert, die außerhalb lebender Organismen durchgeführt werden. In-vitro-Studien, abgeleitet von dem lateinischen Begriff "in Glas", beinhalten Experimente, die in kontrollierten Laborumgebungen unter Verwendung von Zellen oder Geweben durchgeführt werden. Es ist wichtig zu beachten, dass diese Produkte nicht als Arzneimittel oder Medikamente eingestuft sind und keine Zulassung der FDA für die Vorbeugung, Behandlung oder Heilung von medizinischen Zuständen, Beschwerden oder Krankheiten erhalten haben. Wir müssen betonen, dass jede Form der körperlichen Einführung dieser Produkte in Menschen oder Tiere gesetzlich strikt untersagt ist. Es ist unerlässlich, sich an diese Richtlinien zu halten, um die Einhaltung rechtlicher und ethischer Standards in Forschung und Experiment zu gewährleisten.