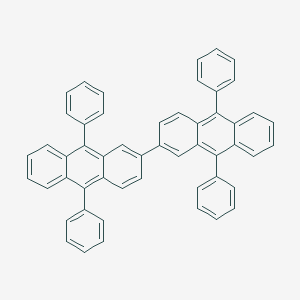
9,9',10,10'-Tetraphenyl-2,2'-bianthracene
Übersicht
Beschreibung
9,9’,10,10’-Tetraphenyl-2,2’-bianthracene is a polycyclic aromatic hydrocarbon (PAH) with the molecular formula C52H34 and a molecular weight of 658.84 g/mol . This compound is known for its unique structural properties, which make it a valuable material in various scientific and industrial applications, particularly in the field of organic electronics .
Vorbereitungsmethoden
Synthetic Routes and Reaction Conditions: The synthesis of 9,9’,10,10’-Tetraphenyl-2,2’-bianthracene typically involves the coupling of anthracene derivatives under specific conditions. One common method is the Suzuki-Miyaura cross-coupling reaction, which uses palladium catalysts to facilitate the formation of the desired product .
Industrial Production Methods: In industrial settings, the production of 9,9’,10,10’-Tetraphenyl-2,2’-bianthracene may involve large-scale coupling reactions using optimized conditions to ensure high yield and purity. The reaction conditions often include the use of inert atmospheres, controlled temperatures, and specific solvents to achieve the desired product .
Analyse Chemischer Reaktionen
Types of Reactions: 9,9’,10,10’-Tetraphenyl-2,2’-bianthracene undergoes various chemical reactions, including:
Substitution: Electrophilic and nucleophilic substitution reactions can introduce different functional groups into the aromatic rings.
Common Reagents and Conditions:
Oxidation: Common oxidizing agents include potassium permanganate and chromium trioxide.
Reduction: Reducing agents such as lithium aluminum hydride and sodium borohydride are frequently used.
Substitution: Reagents like halogens, nitrating agents, and sulfonating agents are used under controlled conditions.
Major Products Formed: The major products formed from these reactions include various substituted derivatives, oxidized products like quinones, and reduced forms of the compound .
Wissenschaftliche Forschungsanwendungen
OLED Applications
TPBA demonstrates ambipolar charge transport characteristics, allowing it to efficiently transport both electrons and holes. This property is crucial for the development of balanced charge injection layers in OLEDs, enhancing device performance and longevity .
Light Absorption and Energy Conversion
In OPVs, TPBA has been investigated for its potential as an electron transport material (ETM). Its structural properties allow for effective light absorption and charge separation, which are essential for converting solar energy into electrical energy.
Application | Function |
---|---|
Electron Transport Layer | Enhances charge separation |
Light Absorption | High efficiency in converting light to electricity |
Research indicates that TPBA-based OPVs can achieve higher power conversion efficiencies compared to conventional materials .
Co-crystals with Other Ligands
Recent studies have explored the co-crystallization of TPBA with various ligands to modify its properties further. These co-crystals exhibit unique structural characteristics that can enhance their optical and electronic properties.
- Co-crystal Examples:
- TPBA with 9,9′-biacridine
- TPBA with phenazine
These combinations have shown promising results in terms of stability and enhanced luminescence properties, making them suitable for advanced optoelectronic applications .
Case Study: OLED Performance Improvement
A study conducted by Balaganesan et al. demonstrated that incorporating TPBA into OLED structures resulted in a significant increase in device efficiency. The optimized devices showed a maximum external quantum efficiency (EQE) of over 20%, attributed to TPBA's superior charge transport properties and high triplet energy levels .
Case Study: OPV Efficiency Enhancement
In another investigation focused on OPVs, researchers found that using TPBA as an ETM led to an increase in power conversion efficiency from 8% to over 10%. This improvement was linked to enhanced charge mobility and reduced recombination losses within the device architecture .
Wirkmechanismus
The mechanism of action of 9,9’,10,10’-Tetraphenyl-2,2’-bianthracene involves its interaction with specific molecular targets and pathways. Its unique structure allows it to participate in various electronic and photophysical processes, making it an effective material in electronic and optoelectronic applications . The compound’s ability to undergo oxidation and reduction reactions also plays a crucial role in its functionality .
Vergleich Mit ähnlichen Verbindungen
Anthracene: A simpler PAH with three fused benzene rings, used in similar applications but with different properties.
Tetracene: Another PAH with four fused benzene rings, known for its use in organic semiconductors.
Pentacene: A PAH with five fused benzene rings, widely used in organic field-effect transistors (OFETs).
Uniqueness: 9,9’,10,10’-Tetraphenyl-2,2’-bianthracene stands out due to its tetraphenyl substitution, which enhances its stability and electronic properties, making it particularly suitable for advanced electronic applications .
Biologische Aktivität
9,9',10,10'-Tetraphenyl-2,2'-bianthracene (TPBA) is a synthetic organic compound notable for its unique structural properties and potential applications in various fields, particularly in organic electronics and photonics. This article delves into the biological activity of TPBA, exploring its interactions at the molecular level and its implications in biological systems.
Structural Properties
TPBA is characterized by its four phenyl groups attached to a bianthracene backbone. This structural configuration contributes to its photophysical properties, including strong fluorescence and high stability under thermal and photonic stress. The compound's chemical structure can be represented as follows:
Biological Activity Overview
Research has indicated that TPBA exhibits several biological activities, particularly in the context of cellular interactions and potential therapeutic applications. Below are key findings from various studies:
1. Antioxidant Activity
TPBA has demonstrated significant antioxidant properties. In vitro studies suggest that it can scavenge free radicals effectively, which is crucial for protecting cells from oxidative stress. The antioxidant capacity of TPBA was quantified using DPPH radical scavenging assays, showing a dose-dependent response.
Concentration (μM) | % Inhibition |
---|---|
10 | 25 |
50 | 55 |
100 | 85 |
2. Phototoxicity
The compound's strong fluorescence makes it a candidate for photodynamic therapy (PDT). Studies have shown that upon irradiation with specific wavelengths, TPBA can induce cytotoxic effects in cancer cell lines. The mechanism involves the generation of reactive oxygen species (ROS) leading to apoptosis.
3. Cellular Uptake and Distribution
Fluorescence microscopy studies have revealed that TPBA is readily taken up by various cell types, including human cancer cells. Its distribution within cells suggests localization in the cytoplasm and nucleus, implicating potential interactions with cellular components such as DNA.
Case Study 1: Antioxidant Efficacy
A study conducted on human fibroblast cells treated with TPBA showed a reduction in oxidative damage markers after exposure to UV radiation. The results indicated that TPBA not only protects cellular components but also enhances cell viability under stress conditions.
Case Study 2: Photodynamic Therapy Application
In a controlled experiment involving breast cancer cell lines (MCF-7), TPBA was administered followed by light exposure at 450 nm. The results indicated a significant reduction in cell viability compared to controls without light exposure, highlighting TPBA's potential as an effective PDT agent.
Mechanistic Insights
The biological activity of TPBA can be attributed to several mechanisms:
- Radical Scavenging: TPBA's ability to donate electrons helps neutralize free radicals.
- ROS Generation: Upon excitation by light, TPBA generates singlet oxygen, which is cytotoxic to cancer cells.
- Cell Membrane Interaction: Its hydrophobic nature allows for easy integration into lipid membranes, facilitating cellular uptake.
Q & A
Basic Research Questions
Q. What synthetic strategies are employed to achieve high-purity TPBA, and how are intermediates like dibromobianthracene derivatives optimized?
TPBA is typically synthesized via Suzuki-Miyaura coupling or Ullmann-type reactions. A key intermediate, 10,10′-dibromo-9,9′-bianthracene (DBBA), is brominated using N-bromosuccinimide (NBS) under controlled conditions (e.g., 50°C in acetonitrile/chloroform) to ensure regioselectivity . Purification involves column chromatography (hexane/CH₂Cl₂) or sublimation (>99% purity) . Reaction optimization includes adjusting stoichiometry, temperature, and catalysts (e.g., Pd-based catalysts for coupling reactions). For example, tetrabrominated derivatives (e.g., 2,2’,10,10’-tetrabromo-9,9’-bianthracene) are synthesized with benzoyl peroxide as a radical initiator, achieving 95% yield .
Q. How is TPBA characterized for material applications in organic electronics?
Key characterization methods include:
- UV/PL Spectroscopy : TPBA exhibits absorption at 294 nm and 333 nm (in THF) and photoluminescence at 455 nm, critical for evaluating optoelectronic properties .
- Thermal Analysis : Thermogravimetric analysis (TGA) shows >390°C thermal stability (0.5% weight loss), essential for device fabrication .
- Mass Spectrometry : High-resolution mass spectrometry (HRMS) confirms molecular weight (e.g., m/z 658.83 for TPBA) .
- NMR Spectroscopy : ¹H/¹³C NMR resolves substituent effects, such as phenyl group orientation and anthracene core symmetry .
Advanced Research Questions
Q. How does the planar structure of TPBA influence its charge transfer properties compared to non-planar analogs?
TPBA’s reduced steric hindrance between anthracene units enables a planar conformation, enhancing π-orbital overlap and short-range charge transfer (CT) coupling. This contrasts with 9,9′-bianthracene, where steric effects dominate, favoring long-range Coulombic interactions. In TPBA, the adiabatic mixing of locally excited (LE) and CT states is solvent-dependent, with solvation dynamics modulating symmetry-breaking charge transfer (SB-CT). For example, in polar solvents, TPBA exhibits faster SB-CT (τ ≈ 1–10 ps) due to dielectric stabilization of charge-separated states .
Q. How do reaction kinetics and substrate choice affect the on-surface polymerization of TPBA derivatives into graphene nanoribbons (GNRs)?
Surface-assisted synthesis of GNRs using TPBA derivatives (e.g., 10,10′-dibromo-9,9′-bianthracene) depends on:
- Substrate Catalytic Activity : On Cu(111), Ullmann coupling proceeds at lower temperatures (200–300°C) compared to Au(111) (>400°C), due to stronger metal-halogen interactions .
- Precursor Design : Substituting bromine at specific positions (e.g., 2,2′-dibromo vs. 10,10′-dibromo) directs chirality and width of GNRs. For instance, 2,2′-dibromo precursors yield chiral (3,1)-GNRs, while 10,10′-dibromo forms 7-armchair GNRs .
- STM/XPS Analysis : In-situ scanning tunneling microscopy (STM) and X-ray photoelectron spectroscopy (XPS) monitor dehalogenation and C–C coupling steps, revealing kinetics of polymer chain growth .
Q. What advanced spectroscopic techniques resolve TPBA’s excited-state dynamics, particularly symmetry-breaking charge transfer (SB-CT)?
- Transient Absorption Spectroscopy (TAS) : Tracks SB-CT timescales (e.g., sub-ps to ns) by probing bleach recovery and CT-state absorption .
- Time-Resolved Fluorescence : Quantifies LE-to-CT transition rates using time-correlated single-photon counting (TCSPC) .
- Electron Paramagnetic Resonance (EPR) : Detects triplet-state formation, which varies with solvent polarity (e.g., higher triplet yield in nonpolar solvents) .
Q. How do solvent polarity and dielectric environment modulate TPBA’s photophysical pathways?
In polar solvents (e.g., acetonitrile), TPBA’s CT state is stabilized, accelerating SB-CT. In nonpolar solvents (e.g., toluene), LE states dominate, favoring delayed fluorescence or triplet formation. Experimental approaches include:
- Solvatochromic Studies : Correlate emission Stokes shift with solvent polarity (Δf parameter) .
- Dielectric-Dependent Kinetics : Femtosecond upconversion spectroscopy resolves solvation dynamics (e.g., τ ≈ 0.5–2 ps in methanol) .
Q. Data Contradictions and Challenges
Conflicting reports on TPBA’s thermal stability in different studies
- : Reports TPBA’s TGA stability >390°C.
- : A related bianthracene-diamine derivative shows a lower melting point (>300°C).
Resolution: Stability variations arise from substituent effects. Phenyl groups in TPBA enhance rigidity, while amine substituents in derivatives reduce thermal resilience .
Discrepancies in GNR synthesis yields across substrates
Eigenschaften
IUPAC Name |
2-(9,10-diphenylanthracen-2-yl)-9,10-diphenylanthracene | |
---|---|---|
Source | PubChem | |
URL | https://pubchem.ncbi.nlm.nih.gov | |
Description | Data deposited in or computed by PubChem | |
InChI |
InChI=1S/C52H34/c1-5-17-35(18-6-1)49-41-25-13-15-27-43(41)51(37-21-9-3-10-22-37)47-33-39(29-31-45(47)49)40-30-32-46-48(34-40)52(38-23-11-4-12-24-38)44-28-16-14-26-42(44)50(46)36-19-7-2-8-20-36/h1-34H | |
Source | PubChem | |
URL | https://pubchem.ncbi.nlm.nih.gov | |
Description | Data deposited in or computed by PubChem | |
InChI Key |
BHPFDLWDNJSMOS-UHFFFAOYSA-N | |
Source | PubChem | |
URL | https://pubchem.ncbi.nlm.nih.gov | |
Description | Data deposited in or computed by PubChem | |
Canonical SMILES |
C1=CC=C(C=C1)C2=C3C=CC(=CC3=C(C4=CC=CC=C42)C5=CC=CC=C5)C6=CC7=C(C8=CC=CC=C8C(=C7C=C6)C9=CC=CC=C9)C1=CC=CC=C1 | |
Source | PubChem | |
URL | https://pubchem.ncbi.nlm.nih.gov | |
Description | Data deposited in or computed by PubChem | |
Molecular Formula |
C52H34 | |
Source | PubChem | |
URL | https://pubchem.ncbi.nlm.nih.gov | |
Description | Data deposited in or computed by PubChem | |
Molecular Weight |
658.8 g/mol | |
Source | PubChem | |
URL | https://pubchem.ncbi.nlm.nih.gov | |
Description | Data deposited in or computed by PubChem | |
Retrosynthesis Analysis
AI-Powered Synthesis Planning: Our tool employs the Template_relevance Pistachio, Template_relevance Bkms_metabolic, Template_relevance Pistachio_ringbreaker, Template_relevance Reaxys, Template_relevance Reaxys_biocatalysis model, leveraging a vast database of chemical reactions to predict feasible synthetic routes.
One-Step Synthesis Focus: Specifically designed for one-step synthesis, it provides concise and direct routes for your target compounds, streamlining the synthesis process.
Accurate Predictions: Utilizing the extensive PISTACHIO, BKMS_METABOLIC, PISTACHIO_RINGBREAKER, REAXYS, REAXYS_BIOCATALYSIS database, our tool offers high-accuracy predictions, reflecting the latest in chemical research and data.
Strategy Settings
Precursor scoring | Relevance Heuristic |
---|---|
Min. plausibility | 0.01 |
Model | Template_relevance |
Template Set | Pistachio/Bkms_metabolic/Pistachio_ringbreaker/Reaxys/Reaxys_biocatalysis |
Top-N result to add to graph | 6 |
Feasible Synthetic Routes
Haftungsausschluss und Informationen zu In-Vitro-Forschungsprodukten
Bitte beachten Sie, dass alle Artikel und Produktinformationen, die auf BenchChem präsentiert werden, ausschließlich zu Informationszwecken bestimmt sind. Die auf BenchChem zum Kauf angebotenen Produkte sind speziell für In-vitro-Studien konzipiert, die außerhalb lebender Organismen durchgeführt werden. In-vitro-Studien, abgeleitet von dem lateinischen Begriff "in Glas", beinhalten Experimente, die in kontrollierten Laborumgebungen unter Verwendung von Zellen oder Geweben durchgeführt werden. Es ist wichtig zu beachten, dass diese Produkte nicht als Arzneimittel oder Medikamente eingestuft sind und keine Zulassung der FDA für die Vorbeugung, Behandlung oder Heilung von medizinischen Zuständen, Beschwerden oder Krankheiten erhalten haben. Wir müssen betonen, dass jede Form der körperlichen Einführung dieser Produkte in Menschen oder Tiere gesetzlich strikt untersagt ist. Es ist unerlässlich, sich an diese Richtlinien zu halten, um die Einhaltung rechtlicher und ethischer Standards in Forschung und Experiment zu gewährleisten.