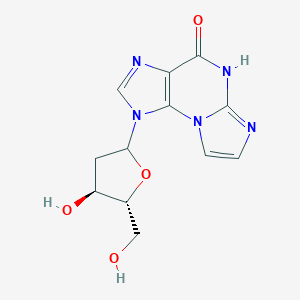
N(2),3-Ethenodeoxyguanosine
Übersicht
Beschreibung
N(2),3-Ethenodeoxyguanosine (N2,3-εdG) is a mutagenic DNA adduct characterized by an etheno bridge linking the N2 and C3 positions of deoxyguanosine . This adduct arises from endogenous and exogenous sources, including:
- Lipid peroxidation: Reactive aldehydes like trans-4-hydroxy-2-nonenal (HNE) form N2,3-εdG via epoxide intermediates under oxidative stress .
- Chemical exposure: Vinyl chloride metabolism generates chloroethylene oxide, which reacts with DNA to produce N2,3-εdG as a minor but persistent adduct .
N2,3-εdG disrupts Watson-Crick base pairing, adopting a syn conformation that promotes mismatches (e.g., pairing with deoxyadenosine), leading to replication errors and genomic instability . Its persistence in vivo is attributed to slower repair rates compared to other etheno adducts .
Vorbereitungsmethoden
Synthetic Routes and Reaction Conditions: N(2),3-Ethenodeoxyguanosine can be synthesized through the reaction of deoxyguanosine with chloroacetaldehyde under slightly alkaline conditions . The reaction typically involves the formation of an unstable intermediate, which is then converted to the desired product. The process requires careful control of pH to prevent degradation of the glycosyl bond .
Industrial Production Methods: The use of α-halocarbonyl compounds and controlled reaction environments are key factors in industrial synthesis .
Analyse Chemischer Reaktionen
Types of Reactions: N(2),3-Ethenodeoxyguanosine undergoes various chemical reactions, including:
Common Reagents and Conditions:
Oxidizing Agents: Hydrogen peroxide and other peroxides are commonly used oxidizing agents.
Nucleophiles: Ammonia and amines are typical nucleophiles that react with this compound.
Major Products: The major products formed from these reactions include various oxidized and substituted derivatives of this compound, which can be further studied for their biological effects .
Wissenschaftliche Forschungsanwendungen
Induction of Mutations
Research has demonstrated that N2,3-εG is a significant contributor to the mutational landscape associated with VC exposure. In various studies, including those utilizing next-generation sequencing techniques, N2,3-εG was shown to induce G to A transitions effectively. This mutation pattern is particularly relevant in the context of VC-associated tumors, where similar mutations have been documented in the Ki-ras proto-oncogene .
Persistence and Repair Mechanisms
One of the critical features of N2,3-εG is its resistance to repair by the AlkB enzyme system, which typically removes similar lesions from DNA. This inability to be repaired contributes to its persistence within cells and tissues, leading to prolonged exposure and higher mutation rates . Studies have indicated that N2,3-εG can remain in rat tissues for extended periods (e.g., 150 days in liver), underscoring its potential role in carcinogenesis .
Analytical Techniques for Detection
To quantify N2,3-εG levels in biological samples, advanced analytical techniques such as gas chromatography coupled with high-resolution mass spectrometry (GC-HRMS) have been developed. These methods allow for sensitive detection of this adduct even at low concentrations, facilitating studies on its formation and effects following carcinogenic exposure .
Drug Development
The unique properties of N2,3-εG have prompted investigations into its potential applications in drug development. For instance, stabilized analogs of N2,3-εG have been synthesized to explore their incorporation into oligonucleotides for targeted mutagenesis studies . These analogs can provide insights into the mechanisms of DNA repair and replication fidelity.
Biomarkers for Exposure Assessment
Given its association with VC exposure and carcinogenesis, N2,3-εG may serve as a biomarker for assessing exposure to environmental carcinogens. The quantification of this adduct in biological samples could help elucidate the relationship between exposure levels and cancer risk.
Case Studies and Research Findings
Study | Findings | Methodology |
---|---|---|
Cheng et al. (2015) | Identified G to A mutation frequency of 13% for N2,3-εG in E. coli | Next-generation sequencing |
Kwon et al. (2016) | Demonstrated that N2,3-εG cannot be repaired by AlkB; linked persistence to mutagenic potential | In vivo analysis using stabilized analogs |
Singer et al. (1987) | Investigated the formation of ethenonucleosides and their role in mutagenesis | Synthesis and analysis of nucleoside derivatives |
Wirkmechanismus
N(2),3-Ethenodeoxyguanosine exerts its effects primarily through the formation of DNA adducts, which can lead to mutations during DNA replication . The compound interacts with DNA polymerases, causing misincorporation of nucleotides and resulting in mutagenesis . The primary molecular targets include the DNA itself and the enzymes involved in DNA replication and repair .
Vergleich Mit ähnlichen Verbindungen
Comparison with Similar Compounds
Structural and Functional Differences
Table 1: Key Properties of Etheno and Propano DNA Adducts
Key Observations:
- Etheno vs. Propano Adducts: Etheno adducts (e.g., N2,3-εdG, 1,N6-εdA) feature a 5-membered ring, while PdG has a 6-membered propano bridge. This structural difference impacts repair efficiency; PdG is resistant to glycosylases, unlike etheno adducts .
- Adduct Formation Pathways: N2,3-εdG forms via epoxidation of lipid peroxidation products (e.g., HNE) or vinyl chloride metabolites . PdG arises from direct Michael addition of HNE to deoxyguanosine in the absence of light .
Repair Mechanisms and Persistence
- N2,3-εdG : Recognized by 3-methyladenine DNA glycosylases (e.g., AlkA, AAG) but repaired slowly, leading to accumulation in tissues .
- 1,N6-εdA : Repaired more efficiently than N2,3-εdG, resulting in lower steady-state levels despite higher initial formation .
- PdG: Not excised by glycosylases, requiring nucleotide excision repair (NER) for removal .
Table 2: Repair Efficiency of Select Adducts
Biomarker and Detection Methods
- N2,3-εdG: Monoclonal antibodies enable sensitive detection in cells exposed to chloroacetaldehyde or lipid peroxidation products .
- 1,N2-Ethenodeoxyguanosine: Forms under specific reaction conditions (light-exposed THF) and serves as a surrogate marker for HNE-induced DNA damage .
Research Findings and Implications
- Vinyl Chloride Carcinogenicity: N2,3-εdG contributes to hepatic angiosarcoma by inducing G→A transitions, a hallmark mutation in vinyl chloride-exposed tissues .
- Lipid Peroxidation Link : Elevated N2,3-εdG levels in colon and liver tissues correlate with diseases like cirrhosis and cancer, highlighting its role in oxidative stress-driven pathologies .
- Therapeutic Insights: Inhibitors of etheno adduct formation (e.g., antioxidants) or enhanced repair pathways may mitigate mutagenesis .
Biologische Aktivität
N(2),3-Ethenodeoxyguanosine (N(2),3-ϵdG) is a significant DNA adduct formed primarily through the reaction of guanine with electrophilic species, notably those derived from vinyl chloride, a known carcinogen. This compound has garnered attention due to its potential mutagenic effects and implications in carcinogenesis. This article explores the biological activity of N(2),3-ϵdG, including its synthesis, mutagenicity, replication mechanisms, and repair pathways.
N(2),3-ϵdG is synthesized through the alkylation of guanosine or deoxyguanosine by vinyl chloride derivatives. The reaction typically involves the formation of an exocyclic double bond that alters the base pairing properties of guanine. The stability and reactivity of N(2),3-ϵdG are influenced by its chemical structure, which includes an additional five-membered ring that can affect its interaction with DNA polymerases.
2.1 Mutagenic Potential
N(2),3-ϵdG is recognized for its mutagenic properties, primarily leading to G to A transitions during DNA replication. Studies have shown that this adduct can cause miscoding by various DNA polymerases:
- Replication Studies : In vitro studies using human Y-family DNA polymerases revealed that polymerases η and κ can replicate through N(2),3-ϵdG, while others like polymerase ι show limited incorporation (one base) opposite the lesion .
- Mutation Frequencies : An indirect assay in Escherichia coli indicated a mutation frequency of approximately 13% for N(2),3-ϵdG, predominantly resulting in G to A transitions .
2.2 Persistence in Cells
The persistence of N(2),3-ϵdG in cells is notable; it has a long half-life in rat liver and lung tissues (approximately 150 days) and in kidney tissues (75 days). This suggests inefficient repair mechanisms for this DNA lesion . The accumulation of such lesions may contribute significantly to the carcinogenic process associated with vinyl chloride exposure.
3.1 DNA Polymerase Interactions
Research indicates that different DNA polymerases exhibit varying efficiencies when replicating past N(2),3-ϵdG:
DNA Polymerase | Incorporation Efficiency | Notes |
---|---|---|
Polymerase η | High | Efficiently incorporates nucleotides opposite N(2),3-ϵdG |
Polymerase κ | Moderate | Can bypass but with lower fidelity |
Polymerase ι | Low | Limited incorporation (1-base) |
REV1 | Low | Similar behavior as polymerase ι |
The structural basis for these interactions has been studied, revealing that polymerase η can accommodate the bulky adduct more effectively than others due to its flexible active site .
4. Repair Mechanisms
The repair of N(2),3-ϵdG lesions is critical for maintaining genomic stability. Human glycosylases involved in base excision repair have been shown to release N(2),3-ϵdG at a significantly slower rate compared to other lesions such as 1,N6-ϵA and 3,N4-ϵC . This inefficiency contributes to the mutagenic potential of the adduct.
5.1 Cancer Associations
Epidemiological studies have linked vinyl chloride exposure to increased cancer risk, particularly liver angiosarcoma. The presence of etheno adducts like N(2),3-ϵdG in tissues from exposed individuals suggests a direct role in tumorigenesis .
5.2 Next-Generation Sequencing Insights
Recent advancements in sequencing technologies have allowed for more detailed examinations of the biological significance of N(2),3-ϵdG lesions in vivo. These studies reveal that etheno DNA adducts are prevalent and contribute significantly to mutational landscapes observed in cancers associated with vinyl chloride exposure .
Q & A
Basic Research Questions
Q. How can N(2),3-Ethenodeoxyguanosine be reliably detected in DNA samples from oxidative stress models?
Methodological Answer: Detection requires sensitive analytical techniques such as:
- Liquid Chromatography-Mass Spectrometry (LC-MS): Enables quantification at femtomolar levels with isotope-labeled internal standards for calibration .
- Immunoassays with Monoclonal Antibodies: Antibodies specific to this compound (e.g., developed via hybridoma technology) allow selective detection in cellular DNA. Cross-reactivity with structurally similar adducts (e.g., 1,N6-ethenodeoxyadenosine) must be ruled out via competitive ELISA .
- 32P-Postlabeling: Useful for low-abundance adducts but requires optimization to avoid interference from endogenous nucleotides .
Q. What experimental models are appropriate for studying endogenous formation mechanisms of this compound?
Methodological Answer:
- In Vitro Peroxidation Systems: Incubate deoxyguanosine or DNA with lipid peroxidation (LPO) products (e.g., 4-hydroxynonenal) under controlled oxidative conditions (e.g., Fe²⁺/H₂O₂). Monitor adduct formation via LC-MS .
- Vinyl Chloride Exposure Models: Treat mammalian cell lines (e.g., HeLa) or rodents with vinyl chloride metabolites (e.g., chloroethylene oxide) to simulate environmental carcinogen exposure. Compare adduct levels using immunoaffinity chromatography .
Q. What are the primary mutagenic consequences of this compound in DNA replication studies?
Methodological Answer:
- In Vitro Replication Assays: Use polymerase fidelity assays (e.g., Klenow fragment) to quantify misincorporation rates. This compound primarily induces GC→AT transitions due to impaired Watson-Crick base pairing .
- E. coli Mutation Reporter Systems: Clone adduct-containing oligonucleotides into plasmids and transform into repair-deficient strains (e.g., alkA tag mutants). Sequence progeny to identify mutation spectra .
Q. How do researchers validate the specificity of this compound antibodies in immunohistochemical studies?
Methodological Answer:
- Competitive Inhibition Assays: Pre-incubate antibodies with excess synthetic this compound to confirm signal reduction.
- Negative Controls: Use tissues from knockout models lacking key oxidative enzymes (e.g., CYP2E1⁻/⁻ mice) to verify baseline adduct levels .
Q. What are the recommended protocols for stabilizing this compound in DNA during extraction?
Methodological Answer:
- DNA Stabilization: Add antioxidants (e.g., deferoxamine) to lysis buffers to prevent artifactual adduct formation during extraction.
- Storage Conditions: Store DNA at -80°C in EDTA-containing buffers to inhibit nuclease activity and metal-catalyzed oxidation .
Advanced Research Questions
Q. How do repair enzyme kinetics influence the interpretation of this compound adduct levels in mutagenicity studies?
Methodological Answer:
- Repair Pathway Inhibition: Treat cells with inhibitors of base excision repair (BER) enzymes (e.g., methoxyamine for AP endonuclease blockade). Quantify adduct persistence and mutation frequency via comet assays or sequencing .
- Kinetic Modeling: Use Michaelis-Menten parameters (e.g., Kₘ and Vₘₐₓ) for repair enzymes (e.g., ALKBH2) to predict adduct half-life in different genomic regions .
Q. What methodological considerations resolve discrepancies in reported GC→AT transition frequencies caused by this compound?
Methodological Answer:
- Context-Specific Analysis: Embed adducts in varying sequence contexts (e.g., CpG vs. non-CpG sites) to assess steric and electronic effects on polymerase misincorporation .
- Single-Molecule Sequencing: Use PacBio SMRT or nanopore sequencing to detect low-frequency mutations masked by bulk assays .
Q. What are the key challenges in synthesizing stable this compound derivatives for structural studies?
Methodological Answer:
- Glycosidic Bond Stability: Protect the deoxyribose moiety with tert-butyldiphenylsilyl (tBDPS) groups during synthesis to prevent hydrolysis. Confirm purity via NOESY NMR to verify β-configuration .
- Deprotection Optimization: Use tetrabutylammonium fluoride (TBAF) under mild acidic conditions (acetic acid/THF) to avoid adduct decomposition .
Q. How can researchers differentiate this compound adducts from other etheno-DNA lesions in complex biological samples?
Methodological Answer:
- Multi-Dimensional Chromatography: Employ tandem LC-MS/MS with orthogonal separation phases (e.g., HILIC followed by reverse-phase) to resolve co-eluting adducts .
- Isotopic Labeling: Synthesize ¹⁵N/¹³C-labeled internal standards for precise quantification and fragmentation pattern matching .
Q. What experimental designs address conflicting data on this compound’s role in hepatocarcinogenesis?
Methodological Answer:
- Integrated Omics Approaches: Combine DNA adduct quantitation with transcriptomic profiling (e.g., RNA-seq) in liver tissues to correlate adduct levels with oncogenic pathway activation .
- In Vivo Repair Knockout Models: Use hepatocyte-specific Aag or Ogg1 knockout mice to isolate the contribution of repair deficiencies to adduct-driven mutagenesis .
Eigenschaften
IUPAC Name |
1-[(4S,5R)-4-hydroxy-5-(hydroxymethyl)oxolan-2-yl]-5H-imidazo[2,1-b]purin-4-one | |
---|---|---|
Source | PubChem | |
URL | https://pubchem.ncbi.nlm.nih.gov | |
Description | Data deposited in or computed by PubChem | |
InChI |
InChI=1S/C12H13N5O4/c18-4-7-6(19)3-8(21-7)17-5-14-9-10(20)15-12-13-1-2-16(12)11(9)17/h1-2,5-8,18-19H,3-4H2,(H,13,15,20)/t6-,7+,8?/m0/s1 | |
Source | PubChem | |
URL | https://pubchem.ncbi.nlm.nih.gov | |
Description | Data deposited in or computed by PubChem | |
InChI Key |
DUHWHZGZQYBCAZ-KJFJCRTCSA-N | |
Source | PubChem | |
URL | https://pubchem.ncbi.nlm.nih.gov | |
Description | Data deposited in or computed by PubChem | |
Canonical SMILES |
C1C(C(OC1N2C=NC3=C2N4C=CN=C4NC3=O)CO)O | |
Source | PubChem | |
URL | https://pubchem.ncbi.nlm.nih.gov | |
Description | Data deposited in or computed by PubChem | |
Isomeric SMILES |
C1[C@@H]([C@H](OC1N2C=NC3=C2N4C=CN=C4NC3=O)CO)O | |
Source | PubChem | |
URL | https://pubchem.ncbi.nlm.nih.gov | |
Description | Data deposited in or computed by PubChem | |
Molecular Formula |
C12H13N5O4 | |
Source | PubChem | |
URL | https://pubchem.ncbi.nlm.nih.gov | |
Description | Data deposited in or computed by PubChem | |
DSSTOX Substance ID |
DTXSID70923651 | |
Record name | 1-(2-Deoxypentofuranosyl)-1H-imidazo[2,1-b]purin-4(5H)-one | |
Source | EPA DSSTox | |
URL | https://comptox.epa.gov/dashboard/DTXSID70923651 | |
Description | DSSTox provides a high quality public chemistry resource for supporting improved predictive toxicology. | |
Molecular Weight |
291.26 g/mol | |
Source | PubChem | |
URL | https://pubchem.ncbi.nlm.nih.gov | |
Description | Data deposited in or computed by PubChem | |
CAS No. |
121055-53-6 | |
Record name | N(2),3-Ethenodeoxyguanosine | |
Source | ChemIDplus | |
URL | https://pubchem.ncbi.nlm.nih.gov/substance/?source=chemidplus&sourceid=0121055536 | |
Description | ChemIDplus is a free, web search system that provides access to the structure and nomenclature authority files used for the identification of chemical substances cited in National Library of Medicine (NLM) databases, including the TOXNET system. | |
Record name | 1-(2-Deoxypentofuranosyl)-1H-imidazo[2,1-b]purin-4(5H)-one | |
Source | EPA DSSTox | |
URL | https://comptox.epa.gov/dashboard/DTXSID70923651 | |
Description | DSSTox provides a high quality public chemistry resource for supporting improved predictive toxicology. | |
Retrosynthesis Analysis
AI-Powered Synthesis Planning: Our tool employs the Template_relevance Pistachio, Template_relevance Bkms_metabolic, Template_relevance Pistachio_ringbreaker, Template_relevance Reaxys, Template_relevance Reaxys_biocatalysis model, leveraging a vast database of chemical reactions to predict feasible synthetic routes.
One-Step Synthesis Focus: Specifically designed for one-step synthesis, it provides concise and direct routes for your target compounds, streamlining the synthesis process.
Accurate Predictions: Utilizing the extensive PISTACHIO, BKMS_METABOLIC, PISTACHIO_RINGBREAKER, REAXYS, REAXYS_BIOCATALYSIS database, our tool offers high-accuracy predictions, reflecting the latest in chemical research and data.
Strategy Settings
Precursor scoring | Relevance Heuristic |
---|---|
Min. plausibility | 0.01 |
Model | Template_relevance |
Template Set | Pistachio/Bkms_metabolic/Pistachio_ringbreaker/Reaxys/Reaxys_biocatalysis |
Top-N result to add to graph | 6 |
Feasible Synthetic Routes
Haftungsausschluss und Informationen zu In-Vitro-Forschungsprodukten
Bitte beachten Sie, dass alle Artikel und Produktinformationen, die auf BenchChem präsentiert werden, ausschließlich zu Informationszwecken bestimmt sind. Die auf BenchChem zum Kauf angebotenen Produkte sind speziell für In-vitro-Studien konzipiert, die außerhalb lebender Organismen durchgeführt werden. In-vitro-Studien, abgeleitet von dem lateinischen Begriff "in Glas", beinhalten Experimente, die in kontrollierten Laborumgebungen unter Verwendung von Zellen oder Geweben durchgeführt werden. Es ist wichtig zu beachten, dass diese Produkte nicht als Arzneimittel oder Medikamente eingestuft sind und keine Zulassung der FDA für die Vorbeugung, Behandlung oder Heilung von medizinischen Zuständen, Beschwerden oder Krankheiten erhalten haben. Wir müssen betonen, dass jede Form der körperlichen Einführung dieser Produkte in Menschen oder Tiere gesetzlich strikt untersagt ist. Es ist unerlässlich, sich an diese Richtlinien zu halten, um die Einhaltung rechtlicher und ethischer Standards in Forschung und Experiment zu gewährleisten.