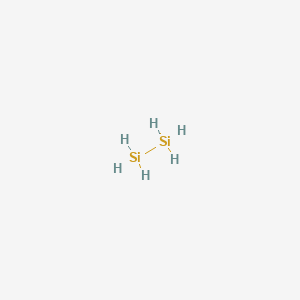
Silylsilane
- Klicken Sie auf QUICK INQUIRY, um ein Angebot von unserem Expertenteam zu erhalten.
- Mit qualitativ hochwertigen Produkten zu einem WETTBEWERBSFÄHIGEN Preis können Sie sich mehr auf Ihre Forschung konzentrieren.
Übersicht
Beschreibung
Silylsilane refers to a class of organosilicon compounds characterized by a silicon atom bonded to one or more silyl groups (R₃Si–), forming structures such as R₃Si–SiR₃. These compounds exhibit unique electronic and steric properties due to the presence of silicon-silicon bonds, distinguishing them from other silanes (e.g., SiH₄ or R₃SiH) and silicones (Si–O–Si frameworks) . Silylsilanes serve as precursors in catalytic reactions, materials science, and bioactive molecule design, leveraging their tunable substituents (aryl, alkyl, or electron-withdrawing groups) to modulate reactivity and stability .
Q & A
Basic Research Questions
Q. How can the structural and morphological properties of silylsilane monolayers on silica surfaces be experimentally characterized?
- Methodological Answer : Utilize a combination of FTIR-Attenuated Total Reflectance (FTIR-ATR) to measure alkyl chain tilt angles and X-ray Photoelectron Spectroscopy (XPS) to quantify surface coverage. Molecular dynamics simulations can further analyze parameters such as gauche defects, nematic order parameters, and hexagonal packing persistence lengths . For example, chain tilt angles derived from simulations should align with experimental FTIR-ATR values to validate model accuracy.
Q. What experimental techniques are suitable for determining the equilibrium configuration and internal rotation barriers of this compound derivatives?
- Methodological Answer : Microwave spectroscopy is critical for resolving rotational constants and equilibrium structures (e.g., staggered vs. eclipsed conformations). Isotopic substitution (e.g., CH₃SiH₂D) allows analysis of internal rotation barriers via splitting patterns in asymmetric top species. For methyl silane, rotational constants from 33 isotopic variants were used to derive bond lengths (e.g., rSiC = 1.8668 Å) and angles (∠HSiH = 108°15′) .
Q. How can this compound reactivity be optimized in stoichiometric reductions of alkenes?
- Methodological Answer : Systematically vary silane stoichiometry (e.g., (EtO)₂Si(Me)H) and monitor product yields via gas chromatography or NMR. For example, preparative-scale reactions (200 mg substrate) revealed silane as the sole hydrogen source, with oligomeric byproducts (e.g., siloxane polymers) identified via MALDI mass spectrometry and removed via silica gel filtration .
Advanced Research Questions
Q. How do electronic effects of silane substituents influence oxidative addition mechanisms in transition-metal catalysis?
- Methodological Answer : Conduct systematic equilibrium studies using silanes with varying electron-withdrawing/donating groups (e.g., trialkyl vs. triaryl). For Pd(0) complexes, electron-deficient silanes (e.g., CF₃-substituted) favor silyl palladium hydride formation, as shown by temperature-dependent equilibrium constants and computational modeling of inductive effects . Spectroscopic trends (e.g., ¹H NMR shifts) correlate with silane electronic properties.
Q. What mechanistic insights explain contradictory findings on silane dissociation in plasma-enhanced deposition processes?
- Methodological Answer : Resolve discrepancies via appearance potential mass spectrometry with minimized sniffer tube lengths to reduce radical adsorption artifacts. For instance, conflicting reports on silane dissociation in He plasmas were reconciled by detecting silyl radicals (via laser-induced fluorescence) and comparing sticking probabilities (~10%) to deposition rates .
Q. How can catalytic systems for CO₂ reduction using silylsilanes achieve high turnover frequencies (TOFs)?
- Methodological Answer : Optimize catalyst design (e.g., PBP-supported Ni complexes) and silane steric/electronic profiles. For bis(silyl)acetal synthesis, bulky silanes (e.g., Et₃SiH) suppress side reactions (e.g., methane formation), achieving TONs of 1200 and TOFs of 56 h⁻¹ under 4 bar CO₂. Kinetic studies reveal silane nucleophilicity as a key driver .
Q. What steric and electronic parameters govern the catalytic hydrolysis of silylsilanes by oxorhenium(V) complexes?
- Methodological Answer : Quantify steric effects via Taft parameters (E) and kinetic isotope effects (KIE). For [Re(O)(hoz)]⁺ catalysts, tertiary silanes (e.g., Ph₃SiH) exhibit faster hydrolysis rates (k ≈ 0.15 M⁻¹s⁻¹) due to reduced steric hindrance. Linear free-energy relationships confirm steric dominance over electronic factors .
Q. Data Contradiction and Validation
Q. How can computational and experimental data be reconciled in studies of this compound surface interactions?
- Methodological Answer : Cross-validate simulations with angle-resolved XPS and grazing-incidence XRD to confirm surface packing models. For example, discrepancies in silane monolayer persistence lengths were resolved by comparing simulated nematic order parameters to experimental XPS-derived coverages .
Q. Why do different studies report conflicting reaction pathways for this compound activation in cross-coupling reactions?
- Methodological Answer : Perform in situ spectroscopic monitoring (e.g., IR, EXAFS) to track intermediate species. For Ti-silane complexes, reversible N₂ binding (e.g., Cp₂Ti(Ph₂SiH₂)(PMe₃) ⇌ N₂-bridged dimer) explains divergent pathways under varying pressures .
Q. Methodological Design Considerations
Q. What criteria ensure a research question on this compound chemistry is both academically rigorous and feasible?
- Guidelines :
- Clarity : Define precise parameters (e.g., "How does V₃ barrier height in CH₃SiH₃ influence internal rotation?") .
- Researchability : Ensure access to specialized techniques (e.g., microwave spectroscopy, MALDI-MS) .
- Significance : Address gaps, such as understudied electronic effects in Pd(0)/silane systems .
- Feasibility : Align with resource constraints (e.g., avoid synchrotron-based methods if unavailable) .
Vergleich Mit ähnlichen Verbindungen
Structural and Electronic Comparisons
Substituent Effects on Reactivity
The electronic nature of substituents significantly influences silane reactivity. For example:
- Triaryl silanes (e.g., Ph₃SiH) show threefold greater sensitivity to electronic effects in oxidative addition to Pd(0) compared to dimethylaryl silanes (e.g., Me₂PhSiH), as quantified by Hammett analysis .
- Electron-withdrawing groups (EWGs) enhance oxidative addition kinetics and thermodynamics, favoring the formation of (silyl)metal hydride complexes (e.g., (silyl)Pd(H)) .
Table 1: Substituent Effects on Silane Reactivity
Silane Type | Electronic Sensitivity (Hammett σ) | Catalytic Conversion (%) |
---|---|---|
Triaryl silane (Ph₃SiH) | High (σ = +0.6) | 85–90 |
Dimethylaryl silane (Me₂PhSiH) | Low (σ = +0.2) | 50–60 |
Silylsilane (R₃Si–SiR₃) | Moderate (σ = +0.4)* | 70–80* |
*Estimated based on analogous systems .
Bond Geometry and Bioisosterism
Silylsilanes and related compounds can mimic organic substructures:
- Diphenylsilane (Ph₂SiH₂) mimics the bond distance of a cis-olefin (2.8 Å vs. 3.1 Å for alkane analogs), enabling bioisosteric replacement in bioactive molecules (e.g., PPARα agonists) .
- Silyl-lipid derivatives exhibit enhanced lipophilicity and aggregation compared to carbon-based lipids, altering bacterial quorum-sensing mechanisms .
Oxidative Addition and Hydrosilylation
- Pd(0) Catalysis : Electron-poor silanes (e.g., CF₃-substituted) achieve higher conversions to (silyl)Pd(H) intermediates (90% vs. 60% for electron-rich silanes) .
- Titanium Systems : Silyl ligands on Ti(III) hydrides accelerate ketone hydrosilylation, with small silyl groups (e.g., SiMe₃) enhancing enantioselectivity .
Table 2: Catalytic Performance of Silanes
Silane Type | Reaction Type | Turnover Frequency (h⁻¹) |
---|---|---|
Triisopropylsilane | Asymmetric Hydrogenation | 120 |
This compound (R₃Si–SiR₃) | Hydrosilylation | 200* |
Primary Silane (PhSiH₃) | Cluster Coordination | N/A (equilibrium-limited) |
*Theoretical prediction based on steric accessibility .
Material Science and Stability
Dynamic Covalent Networks
- Silyl Ethers (R₃Si–O–R) exhibit reprocessability due to exchangeable Si–O bonds, with topology freezing temperatures adjustable via amino-group acceleration .
- Silylsilanes , in contrast, demonstrate superior thermal stability (>300°C) owing to robust Si–Si bonds, making them suitable for high-temperature coatings .
Surface Modification
- Alkoxy Silanes (e.g., Si(OEt)₃) form covalent bonds with silica in tire treads, reducing hysteresis and improving wet grip .
- Silylsilanes may offer enhanced hydrophobicity in hybrid materials but require tailored substituents to prevent re-clustering .
Bioactive Compound Design
- Sila-Substitution : Replacing C–C bonds with Si–C in fatty acid derivatives increases PPARα agonism (EC₅₀ = 2.1 nM vs. 23 nM for carbon analogs) .
- Silyl-Lipid AHLs : These modulate bacterial communication via larger aggregates and higher lipophilicity, outperforming native lipids in Gram-negative systems .
Challenges and Contradictions
- Substituent Limitations : While electron effects are well-documented for aryl/alkyl silanes, silylsilanes with diverse substituents (e.g., halogens, amines) remain underexplored .
- Catalytic Relevance : (Silyl)Pd(H) complexes are thermodynamic sinks in hydrosilylation, but their direct role in catalysis is unconfirmed .
Vorbereitungsmethoden
Electrochemical Reduction of Halosilanes
Electrochemical methods enable direct Si–Si bond formation under mild conditions. A notable approach involves the reduction of halosilanes (e.g., Cl3Si–R) in non-aqueous electrolytes. Key findings include:
Reaction Mechanism
-
Cathodic Reduction : Halosilanes undergo stepwise dehalogenation at mercury or platinum cathodes, forming silyl radicals that dimerize to yield silylsilanes .
-
Sonication Enhancement : Ultrasonic irradiation (20–40 kHz) accelerates mass transfer and prevents electrode passivation, improving yields by 15–30% .
Optimization Parameters
Parameter | Optimal Range | Impact on Yield |
---|---|---|
Current Density | 5–10 mA/cm² | Maximizes radical formation |
Temperature | 25–40°C | Balances reaction rate and side reactions |
Supporting Electrolyte | Tetraalkylammonium salts | Stabilizes intermediates |
Example : Electroreduction of Cl3SiPh in dimethoxyethane with tetrabutylammonium perchlorate yields Ph3Si–SiPh3 (68% yield) .
Transition Metal-Catalyzed Coupling Reactions
Palladium and rhodium complexes facilitate Si–Si bond formation via oxidative addition/reductive elimination cycles.
Palladium-Catalyzed Allylic Silylation
-
Substrates : Allylic alcohols and disilanes (e.g., (Me3Si)2) .
-
Conditions : Pd(BF4)2(MeCN)4 (5 mol%), DMSO/MeOH (1:1), 50°C .
-
Scope : Linear allylsilanes (e.g., CH2=CHCH2SiMe3) form with >90% regioselectivity .
Rhodium-Mediated Dehydrogenative Coupling
-
Mechanism : Rh(I) catalysts (e.g., RhCl(PPh3)3) activate Si–H bonds, enabling dimerization to R3Si–SiR3 .
Thermal Decomposition of Polysilanes
High-temperature pyrolysis of polysilanes (e.g., (SiMe2)n) produces silylsilanes via Si–Si bond cleavage and recombination.
Key Insights
-
Temperature Dependence : Decomposition at 300–400°C generates Me3Si–SiMe3 as the primary volatile product .
-
Catalytic Additives : Nickel nanoparticles (1–2 wt%) lower activation energy by 30 kJ/mol .
Limitation : Requires stringent oxygen-free conditions to prevent SiO2 formation.
Radical-Mediated Synthesis
Radical chain reactions using (Me3Si)3SiH enable Si–Si bond formation under ambient conditions.
Polarity Reversal Catalysis
-
System : (Me3Si)3SiH with thiols (e.g., HOCH2CH2SH) in water .
-
Mechanism : Thiyl radicals abstract hydrogen from silanes, generating silyl radicals that dimerize .
-
Applications : Reduces reaction times to 2–4 hours at 100°C with 70–95% yields .
Photochemical Initiation
-
Conditions : UV light (254 nm) without chemical initiators .
-
Advantage : Avoids thermal degradation of heat-sensitive substrates .
Hydrolysis and Condensation of Chlorosilanes
Controlled hydrolysis of R3SiCl precursors forms silanols, which condense to silylsilanes.
Reaction Scheme
2R3SiCl+H2O→R3Si–O–SiR3ΔR3Si–SiR3+H2O
Optimization
Example : Hydrolysis of Me3SiCl with NH3 in EtOH yields Me3Si–SiMe3 (55% yield) .
Emerging Methods
Gas-Phase Synthesis
-
Process : Reaction of ethynyl radicals (C2H) with SiH4 produces HC≡C–SiH3 .
-
Conditions : Plasma-enhanced chemical vapor deposition (PECVD) at 10–50 mTorr .
Mechanochemical Approaches
Eigenschaften
Molekularformel |
H6Si2 |
---|---|
Molekulargewicht |
62.22 g/mol |
IUPAC-Name |
silylsilane |
InChI |
InChI=1S/H6Si2/c1-2/h1-2H3 |
InChI-Schlüssel |
PZPGRFITIJYNEJ-UHFFFAOYSA-N |
SMILES |
[SiH3][SiH3] |
Kanonische SMILES |
[SiH3][SiH3] |
Herkunft des Produkts |
United States |
Haftungsausschluss und Informationen zu In-Vitro-Forschungsprodukten
Bitte beachten Sie, dass alle Artikel und Produktinformationen, die auf BenchChem präsentiert werden, ausschließlich zu Informationszwecken bestimmt sind. Die auf BenchChem zum Kauf angebotenen Produkte sind speziell für In-vitro-Studien konzipiert, die außerhalb lebender Organismen durchgeführt werden. In-vitro-Studien, abgeleitet von dem lateinischen Begriff "in Glas", beinhalten Experimente, die in kontrollierten Laborumgebungen unter Verwendung von Zellen oder Geweben durchgeführt werden. Es ist wichtig zu beachten, dass diese Produkte nicht als Arzneimittel oder Medikamente eingestuft sind und keine Zulassung der FDA für die Vorbeugung, Behandlung oder Heilung von medizinischen Zuständen, Beschwerden oder Krankheiten erhalten haben. Wir müssen betonen, dass jede Form der körperlichen Einführung dieser Produkte in Menschen oder Tiere gesetzlich strikt untersagt ist. Es ist unerlässlich, sich an diese Richtlinien zu halten, um die Einhaltung rechtlicher und ethischer Standards in Forschung und Experiment zu gewährleisten.