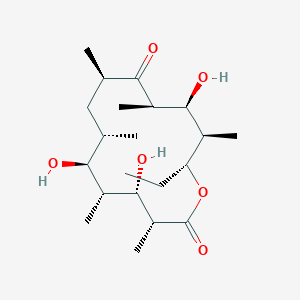
6-deoxyerythronolide B
Übersicht
Beschreibung
6-Deoxyerythronolide B (6-dEB) is the 14-membered macrolactone aglycone precursor to erythromycin antibiotics, a critical class of macrolide antibacterials . Biosynthesized by the modular polyketide synthase (PKS) this compound synthase (DEBS), 6-dEB serves as the foundational scaffold for erythromycin A and B after post-PKS hydroxylation and glycosylation . Its stereochemical complexity—featuring 10 stereocenters—aligns with Celmer’s Rules, a unifying model for macrolide stereochemistry . The compound has been a focal point of synthetic and biosynthetic studies due to its structural intricacy and therapeutic relevance.
Vorbereitungsmethoden
Chemical Synthesis of 6-Deoxyerythronolide B
Total Synthesis via C–C Bond-Forming Transfer Hydrogenation
The Krische laboratory developed a 14-step linear synthesis (20 total steps) leveraging iridium- and ruthenium-catalyzed crotylation for stereocontrol :
Key steps :
-
Fragment A (C7–C13) synthesis :
-
Fragment B (C2–C6) synthesis :
-
Macrocyclization :
Advantages :
Limitations :
Formal Synthesis via Asymmetric Aldol Reactions
Mortison et al. achieved the carbon skeleton in 23 steps using iterative propionate aldol reactions :
Critical transformations :
Comparison to total synthesis :
Parameter | Krische (2013) | Mortison (2006) |
---|---|---|
Longest linear sequence | 14 steps | 23 steps |
Total steps | 20 | 23 |
Key stereochemistry | 9/9 centers | 8/9 centers |
Macrocyclization method | Enyne metathesis | Lactonization |
Heterologous Biosynthesis of this compound
DEBS Enzyme Architecture and Function
The this compound synthase (DEBS) comprises three polypeptides (DEBS1–3) with six modules :
Domain organization :
Module | Domains | Function |
---|---|---|
1 | KS, AT, ACP, KR | Propionate loading and reduction |
2–6 | KS, AT, ACP, KR, DH | Chain elongation and modification |
Catalytic mechanism :
Microbial Production in Escherichia coli
Optimized fed-batch processes achieve 700 mg/L 6-dEB :
Critical parameters :
-
Medium composition : Tryptone supplementation increases DEBS solubility (22-fold titer improvement) .
-
Propionate feeding : 5 mM exogenous propionate enhances methylmalonyl-CoA availability .
Scale-up performance :
Bioreactor scale | Cell density (g/L) | 6-dEB titer (mg/L) | Plasmid retention (%) |
---|---|---|---|
5 L | 30 | 700 | 90–100 |
Microscale | 15 | 160 | 85 |
Challenges :
Comparative Analysis of Preparation Methods
Efficiency Metrics
Method | Step count | Yield (%) | Scalability |
---|---|---|---|
Chemical synthesis | 14 | 57 | Lab-scale |
Formal synthesis | 23 | 12 | Lab-scale |
Heterologous | N/A | 160 mg/L | Industrial |
Strategic Advantages
Analyse Chemischer Reaktionen
Reaktionstypen: 6-Deoxyerythronolide B unterliegt verschiedenen chemischen Reaktionen, darunter Oxidation, Reduktion und Substitution. Eine bemerkenswerte Reaktion ist die NADPH-abhängige Umwandlung von this compound zu Erythronolide B durch die Insertion eines Sauerstoffatoms .
Häufige Reagenzien und Bedingungen: Häufige Reagenzien, die bei der Synthese und Modifikation von this compound verwendet werden, sind Propionyl-CoA, S-Methylmalonyl-CoA und verschiedene Oxidationsmittel. Die Reaktionen erfordern oft spezifische Enzyme wie Polyketidsynthasen und Oxidasen .
Hauptprodukte: Das Hauptprodukt, das bei der Oxidation von this compound entsteht, ist Erythronolide B, ein wichtiges Zwischenprodukt bei der Biosynthese von Erythromycin .
Wissenschaftliche Forschungsanwendungen
Antibiotic Development
Erythromycin Derivatives
6-dEB serves as the aglycone precursor for erythromycin, a widely used antibiotic effective against a range of bacterial infections. The structural modifications of 6-dEB lead to various erythromycin derivatives with enhanced pharmacological properties. For example, the total synthesis of 6-dEB has been achieved using advanced synthetic strategies such as late-stage C–H oxidation, which allows for the efficient construction of complex molecular architectures .
Mechanism of Action
The mechanism by which erythromycin and its derivatives exert their antibacterial effects involves binding to the bacterial ribosome, inhibiting protein synthesis. This action is critical for treating infections caused by Gram-positive bacteria and some Gram-negative bacteria.
Synthetic Chemistry
Total Synthesis Strategies
Recent advancements in synthetic methodologies have facilitated the efficient total synthesis of 6-dEB. For instance, a study reported a total synthesis involving a late-stage C–H oxidative macrolactonization strategy that minimized side reactions and improved overall yield . The following table summarizes various synthetic approaches:
Synthesis Method | Key Features | Yield (%) |
---|---|---|
Late-stage C–H oxidation | High regio- and diastereoselectivity | 7.8 |
C–C bond-forming hydrogenation | Efficient construction of complex molecules | TBD |
Biotechnological Production
Heterologous Expression Systems
The production of 6-dEB has been successfully achieved in various microbial systems, notably in Bacillus subtilis and Escherichia coli. These systems utilize engineered polyketide synthases (PKSs) to produce 6-dEB efficiently. A notable study demonstrated that the expression of the deoxyerythronolide B synthase (DEBS) genes in Bacillus subtilis led to significant yields of 6-dEB when optimized genetic modifications were applied .
Production Strategies
The following table outlines different microbial hosts used for the production of 6-dEB:
Microbial Host | Production Yield | Genetic Modifications |
---|---|---|
Bacillus subtilis | Moderate | Knockout of native secondary metabolite clusters |
Escherichia coli | High | Optimized ribosomal binding sites |
Case Studies
Case Study: Total Synthesis via C–C Bond Formation
A recent study highlighted the total synthesis of 6-dEB using a C–C bond-forming transfer hydrogenation method. This approach demonstrated significant improvements in yield and efficiency compared to traditional methods, showcasing the potential for developing new antibiotics with better efficacy profiles .
Case Study: Heterologous Production Optimization
In another study focusing on Bacillus subtilis, researchers optimized conditions for the secretion of 6-dEB into the medium. By modifying metabolic pathways and utilizing specific promoters, they achieved enhanced production levels, indicating the potential for industrial-scale applications in antibiotic manufacturing .
Wirkmechanismus
The mechanism of action of 6-Deoxyerythronolide B involves its conversion to erythronolide B by specific enzymes. This conversion is crucial for the biosynthesis of erythromycin, which exerts its antibiotic effects by inhibiting bacterial protein synthesis. The molecular targets of erythromycin include the bacterial ribosome, where it binds and prevents the elongation of the peptide chain .
Vergleich Mit ähnlichen Verbindungen
Limitations :
- High cost of diketide-SNAC precursors .
- Substrate toxicity at elevated concentrations, requiring controlled feeding strategies .
Structural Homologues in the Macrolide Family
- Erythronolide B: The hydroxylated derivative of 6-dEB, differing by a C6 hydroxyl group. Its synthesis requires additional oxidative steps, complicating production .
- Pikromycin/Narbonolide: Produced by the pikromycin PKS, these 12- and 14-membered macrolactones share modular PKS architecture with DEBS but diverge in substrate specificity (methylmalonyl-CoA vs. malonyl-CoA) and cyclization logic .
- Epothilone D : A 16-membered macrolide with antitumor activity. While structurally distinct, its biosynthesis involves analogous PKS machinery, highlighting conserved catalytic domains (e.g., ketosynthase, acyltransferase) .
Data Tables
Table 2: Comparison of 6-dEB with Structural Homologues
Compound | Ring Size | Key Modifications | Biosynthetic System | Bioactivity |
---|---|---|---|---|
6-dEB | 14 | None (parent aglycone) | DEBS (modular PKS) | Antibacterial |
Erythronolide B | 14 | C6 hydroxylation | DEBS + P450 hydroxylase | Antibacterial |
Narbonolide | 14 | C12 desmethyl | Pik PKS | Antibacterial |
Epothilone D | 16 | Epoxide, thiazole side chain | Hybrid PKS-NRPS | Antitumor |
Research Findings and Key Differences
- Synthetic Efficiency : Late-stage C–H oxidation (Stang & White, 2009) reduced total synthesis steps to 22, achieving >40:1 diastereoselectivity . Krische’s transfer hydrogenation route further streamlined synthesis to 14 linear steps .
- Domain Specificity in DEBS : The ketosynthase (KS) and acyltransferase (AT) domains are spatially separated (~80 Å), necessitating dynamic repositioning of the acyl carrier protein (ACP) for substrate channeling .
- Host-Dependent Titers : S. coelicolor outperforms E. coli in 6-dEB analogue production (e.g., 371 mg/L vs. 30 mg/L for "propyl-diketide"-SNPC) due to superior precursor uptake and tolerance .
Biologische Aktivität
6-Deoxyerythronolide B (6dEB) is a significant polyketide compound that serves as a precursor in the biosynthesis of erythromycin, a widely used antibiotic. This article delves into its biological activity, production mechanisms, and potential therapeutic applications, supported by relevant case studies and research findings.
Overview of this compound
6dEB is produced by the action of the This compound synthase (DEBS) , a multi-modular polyketide synthase (PKS) found in Saccharopolyspora erythraea. The synthesis involves complex enzymatic reactions that utilize various substrates to construct the polyketide backbone. The compound's structure is critical for its biological activity, particularly its antibiotic properties.
The biological activity of 6dEB primarily stems from its role as a precursor in the synthesis of erythromycin. Erythromycin exhibits broad-spectrum antibacterial activity by inhibiting bacterial protein synthesis. It binds to the 50S ribosomal subunit, preventing peptide bond formation, which effectively halts bacterial growth. The conversion of 6dEB to erythromycin involves hydroxylation at the C-6 position, catalyzed by specific enzymes that require ferredoxin and NADPH as cofactors .
Biological Activities
Antimicrobial Properties
Research indicates that 6dEB and its derivatives possess significant antimicrobial properties. The compound has been shown to exhibit activity against various Gram-positive bacteria, including Staphylococcus aureus and Streptococcus pneumoniae. The effectiveness of 6dEB as an antibiotic is attributed to its structural similarity to erythromycin, allowing it to interfere with bacterial ribosomal function .
Antitumor Activity
Beyond its antibacterial effects, studies have suggested potential antitumor activities associated with 6dEB. Some derivatives have demonstrated cytotoxic effects against cancer cell lines, indicating a broader spectrum of biological activity that warrants further investigation .
Case Study 1: Production Optimization
A study focused on optimizing the production of 6dEB in Bacillus subtilis highlighted genetic modifications that enhanced yield. By knocking out native secondary metabolite clusters, researchers achieved a significant increase in 6dEB production when exogenous propionate was supplied . This study underscores the potential for metabolic engineering to enhance polyketide production.
Case Study 2: Chemobiosynthesis
Another research effort explored chemobiosynthesis methods to produce novel analogs of 6dEB. By modifying the DEBS system and feeding diketide substrates, researchers generated various analogs with improved bioactivity profiles. This approach allows for high-yield production while maintaining structural diversity .
Table 1: Biological Activities of this compound and Derivatives
Table 2: Production Strains for this compound
Q & A
Q. Basic: What is the modular architecture of 6-deoxyerythronolide B synthase (DEBS), and how does it coordinate biosynthesis?
DEBS is a 2-MDa trimeric polyketide synthase (PKS) composed of three homodimers (DEBS1, DEBS2, DEBS3) encoded by the erythromycin biosynthetic gene cluster . Each homodimer contains catalytically independent enzymatic "modules" that sequentially elongate and modify the polyketide chain. Modules are organized into domains: ketosynthase (KS), acyltransferase (AT), ketoreductase (KR), dehydratase (DH), enoylreductase (ER), and acyl carrier protein (ACP). Structural studies using small-angle X-ray scattering (SAXS) and crystallography revealed that domain-domain interactions and nonconserved linker regions mediate intermodular communication .
Q. Basic: What are the key enzymatic domains in DEBS modules, and how do they contribute to 6-dEB biosynthesis?
Each DEBS module contains a KS domain (chain elongation via decarboxylative Claisen condensation), an AT domain (selects extender units like methylmalonyl-CoA), and an ACP domain (shuttles intermediates). Optional reductive domains (KR, DH, ER) control stereochemistry and saturation. For example, KR domains set 9/10 stereocenters in 6-dEB through diastereospecific reductions . The thioesterase (TE) domain in DEBS3 catalyzes cyclization to form the macrolactone . Domain functions are validated via mutagenesis and in vitro reconstitution .
Q. Advanced: How can DEBS be reconstituted in vitro, and what insights does this provide into assembly-line kinetics?
Full DEBS reconstitution involves purifying individual subunits (DEBS1-3) and measuring NADPH consumption (linked to KR/ER activity) via UV spectrophotometry, validated by LC-MS quantification of 6-dEB . Kinetic assays revealed:
- Turnover rates: 1.1 min⁻¹ (hexamodular DEBS) vs. 21 min⁻¹ (bimodular truncations) .
- Substrate promiscuity: DEBS incorporates ethylmalonyl-CoA, yielding 8-ethyl-8-demethyl-6-dEB at non-limiting concentrations .
This system enables mechanistic studies of domain cooperativity and substrate specificity .
Q. Advanced: What experimental strategies address intermodular communication challenges in DEBS engineering?
Intermodular transfer efficiency depends on:
- Linker regions : N-/C-terminal linkers between adjacent modules mediate docking. Swapping linkers alters intermediate channeling .
- ACP-KS interactions : Molecular recognition between donor ACP and acceptor KS domains ensures fidelity. Hybrid PKS systems (e.g., PKS-NRPS) require compatible ACP-KS pairs .
Quantitative assays using chimeric modules (e.g., DEBS module 2 fused to heterologous modules) reveal context-dependent tolerance for non-native substrates .
Q. Advanced: How is stereochemical control achieved in DEBS, and what mutations disrupt this process?
Stereochemistry at C-3, C-5, C-7, C-9, C-11, and C-13 is dictated by KR domains through stereospecific hydride transfer and epimerization . For example:
- KR1 in DEBS module 1 establishes the C-3 R configuration.
- Mutating KR active-site residues (e.g., Tyr → Phe) alters stereochemical outcomes .
Crystallographic studies of KR domains bound to acyl-ACP substrates reveal conserved hydrogen-bonding networks critical for stereocontrol .
Q. Advanced: How do contradictory data on DEBS substrate specificity inform engineering approaches?
While DEBS is selective for methylmalonyl-CoA under physiological conditions, in vitro studies show unexpected tolerance for ethylmalonyl-CoA, producing 8-ethyl-6-dEB analogs . This contradicts earlier assumptions of strict substrate specificity. Resolution strategies include:
- Kinetic profiling : Comparing for native vs. non-native substrates .
- Structural analysis : Engineering KS domains to accommodate bulkier extender units via active-site mutations (e.g., F381A in KS6) .
Q. Advanced: What methodological advances enable heterologous production of 6-dEB in E. coli?
Key steps include:
- Codon optimization : Redesigning DEBS genes for E. coli expression .
- Metabolic engineering : Overproducing methylmalonyl-CoA via mutase/epimerase pathways .
- Dynamic regulation : Using synthetic small RNAs (sRNAs) to fine-tense competing pathways (e.g., fatty acid biosynthesis) .
Fed-batch fermentation with optimized media achieves titers >100 mg/L .
Q. Advanced: How do structural dynamics of DEBS influence catalytic efficiency?
SAXS and cryo-EM studies show DEBS adopts flexible conformations during catalysis:
Eigenschaften
CAS-Nummer |
15797-36-1 |
---|---|
Molekularformel |
C21H38O6 |
Molekulargewicht |
386.5 g/mol |
IUPAC-Name |
(3R,4S,5R,6S,7S,9R,11R,12S,13R,14R)-14-ethyl-4,6,12-trihydroxy-3,5,7,9,11,13-hexamethyl-oxacyclotetradecane-2,10-dione |
InChI |
InChI=1S/C21H38O6/c1-8-16-12(4)19(24)13(5)17(22)10(2)9-11(3)18(23)14(6)20(25)15(7)21(26)27-16/h10-16,18-20,23-25H,8-9H2,1-7H3/t10-,11+,12+,13+,14-,15-,16-,18+,19+,20+/m1/s1 |
InChI-Schlüssel |
HQZOLNNEQAKEHT-IBBGRPSASA-N |
SMILES |
CCC1C(C(C(C(=O)C(CC(C(C(C(C(C(=O)O1)C)O)C)O)C)C)C)O)C |
Isomerische SMILES |
CC[C@@H]1[C@@H]([C@@H]([C@H](C(=O)[C@@H](C[C@@H]([C@@H]([C@H]([C@@H]([C@H](C(=O)O1)C)O)C)O)C)C)C)O)C |
Kanonische SMILES |
CCC1C(C(C(C(=O)C(CC(C(C(C(C(C(=O)O1)C)O)C)O)C)C)C)O)C |
Synonyme |
3,5,11-trihydroxyerythranolid-9-one 6-deoxyerythronolide B |
Herkunft des Produkts |
United States |
Retrosynthesis Analysis
AI-Powered Synthesis Planning: Our tool employs the Template_relevance Pistachio, Template_relevance Bkms_metabolic, Template_relevance Pistachio_ringbreaker, Template_relevance Reaxys, Template_relevance Reaxys_biocatalysis model, leveraging a vast database of chemical reactions to predict feasible synthetic routes.
One-Step Synthesis Focus: Specifically designed for one-step synthesis, it provides concise and direct routes for your target compounds, streamlining the synthesis process.
Accurate Predictions: Utilizing the extensive PISTACHIO, BKMS_METABOLIC, PISTACHIO_RINGBREAKER, REAXYS, REAXYS_BIOCATALYSIS database, our tool offers high-accuracy predictions, reflecting the latest in chemical research and data.
Strategy Settings
Precursor scoring | Relevance Heuristic |
---|---|
Min. plausibility | 0.01 |
Model | Template_relevance |
Template Set | Pistachio/Bkms_metabolic/Pistachio_ringbreaker/Reaxys/Reaxys_biocatalysis |
Top-N result to add to graph | 6 |
Feasible Synthetic Routes
Haftungsausschluss und Informationen zu In-Vitro-Forschungsprodukten
Bitte beachten Sie, dass alle Artikel und Produktinformationen, die auf BenchChem präsentiert werden, ausschließlich zu Informationszwecken bestimmt sind. Die auf BenchChem zum Kauf angebotenen Produkte sind speziell für In-vitro-Studien konzipiert, die außerhalb lebender Organismen durchgeführt werden. In-vitro-Studien, abgeleitet von dem lateinischen Begriff "in Glas", beinhalten Experimente, die in kontrollierten Laborumgebungen unter Verwendung von Zellen oder Geweben durchgeführt werden. Es ist wichtig zu beachten, dass diese Produkte nicht als Arzneimittel oder Medikamente eingestuft sind und keine Zulassung der FDA für die Vorbeugung, Behandlung oder Heilung von medizinischen Zuständen, Beschwerden oder Krankheiten erhalten haben. Wir müssen betonen, dass jede Form der körperlichen Einführung dieser Produkte in Menschen oder Tiere gesetzlich strikt untersagt ist. Es ist unerlässlich, sich an diese Richtlinien zu halten, um die Einhaltung rechtlicher und ethischer Standards in Forschung und Experiment zu gewährleisten.