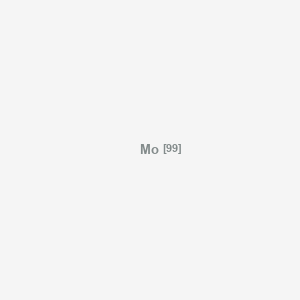
Molybdenum Mo-99
- Klicken Sie auf QUICK INQUIRY, um ein Angebot von unserem Expertenteam zu erhalten.
- Mit qualitativ hochwertigen Produkten zu einem WETTBEWERBSFÄHIGEN Preis können Sie sich mehr auf Ihre Forschung konzentrieren.
Übersicht
Beschreibung
Molybdenum-99 (Mo-99) is a radioactive isotope critical to nuclear medicine, serving as the parent isotope for Technetium-99m (Tc-99m), the most widely used radionuclide in diagnostic imaging. Mo-99 has a half-life of 66 hours and decays via beta emission to Tc-99m, which emits a 140 keV gamma ray ideal for medical imaging . Approximately 80% of nuclear medicine procedures rely on Tc-99m for applications such as cardiac, cancer, and bone diagnostics .
Vorbereitungsmethoden
Reactor-Based Production Methods
Uranium-235 Fission
The predominant method for Mo-99 production involves neutron-induced fission of U-235 in research reactors. When U-235 absorbs a thermal neutron, it undergoes fission, yielding a spectrum of fission products, including Mo-99 with a fission yield of approximately 6.1% . Highly enriched uranium (HEU, >90% U-235) has historically been used due to its high neutron capture cross-section, enabling efficient Mo-99 production with specific activities exceeding 1,000 Ci/g . Post-irradiation, the target is dissolved in nitric acid, and Mo-99 is separated chromatographically using alumina columns or precipitation methods .
Table 1: Comparative Reactor-Based Production Parameters
Parameter | U-235 Fission (HEU) | Neutron Capture (Mo-98) |
---|---|---|
Specific Activity (Ci/g) | >1,000 | 0.1–1 |
Fission Yield (%) | 6.1 | N/A |
Primary Contaminants | I-131, Ru-103 | Stable Mo isotopes |
Infrastructure Required | Nuclear reactor | Nuclear reactor |
Despite its efficiency, HEU use raises proliferation concerns, prompting a shift toward low-enriched uranium (LEU, <20% U-235) under initiatives like the IAEA Coordinated Research Project . LEU targets require larger quantities of uranium and generate more waste but mitigate security risks .
Neutron Capture on Molybdenum-98
An alternative reactor-based approach involves irradiating Mo-98-enriched targets with neutrons, transmuting Mo-98 into Mo-99 via the (n,γ) reaction . While this method avoids uranium handling, the resulting Mo-99 has low specific activity (0.1–1 Ci/g), necessitating post-irradiation isotopic enrichment for medical use. This limits its applicability compared to fission-derived Mo-99 .
Accelerator-Based Production Methods
Charged Particle Bombardment
Recent patents describe Mo-99 production via charged particle (e.g., protons, deuterons) bombardment of thorium-232 (Th-232) or uranium targets in cyclotrons or linear accelerators . At beam energies exceeding 15 MeV, fissile nuclei undergo fission, releasing Mo-99 alongside other products. For instance, a 30 MeV proton beam on Th-232 yields tens of curies of Mo-99, with efficiency scaling linearly with beam intensity (100 μA–100 mA) . Post-irradiation, Mo-99 is separated using ion exchange, electrolysis, or sublimation, and the target material is recycled .
Table 2: Accelerator Beam Parameters for Optimal Mo-99 Yield
Beam Energy (MeV) | Target Material | Beam Current (μA) | Mo-99 Yield (Ci) |
---|---|---|---|
15–30 | Th-232 | 100–100,000 | 10–50 |
>30 | U-238 | 50–50,000 | 20–100 |
Photonuclear Reactions
Experimental methods explore photonuclear reactions, where high-energy photons (γ-rays) from electron accelerators induce fission in uranium targets. Although less established, this approach offers potential for decentralized production without neutron sources .
Chemical Separation and Purification Techniques
Chromatographic Purification
Industrial protocols, such as those by Nordion, dissolve irradiated uranium targets in nitric acid, followed by chromatographic purification on alumina columns . Mo-99 adsorbs as molybdate ions (MoO₄²⁻) at pH 3–4, while fission products like I-131 and Ru-103 are eluted separately. The final product is stabilized in 0.2N NaOH with optional NaOCl addition .
Sublimation and Ion Exchange
The patent EP2398023A1 highlights sublimation as a high-purity separation method, where Mo-99 is volatilized at elevated temperatures and collected on cooled surfaces . Ion exchange resins selectively bind Mo-99, which is later eluted with ammonium nitrate solutions .
Quality Control and Radiopurity Specifications
Nordion’s product specifications mandate rigorous purity standards :
-
Gamma emitters : ≤0.05 μCi/mCi for I-131, Ru-103, and Te-132.
-
Beta emitters : ≤0.0006 μCi/mCi for Sr-89 and ≤0.000015 μCi/mCi for Sr-90.
-
Alpha emitters : ≤1×10⁻⁷ μCi/mCi.
These thresholds ensure patient safety and compliance with pharmacopeial guidelines.
Recent Advances and Alternative Methods
LEU-Based Fission
The IAEA promotes LEU adoption to replace HEU, with small-scale producers achieving comparable yields through optimized target geometries and neutron flux management . For example, the Argentine RA-3 reactor produces 1,200 Ci/week using LEU .
Target Recycling
Cyclotron-driven methods enable target recycling, reducing waste and cost. After Mo-99 extraction, the thorium or uranium target is reconstituted for subsequent irradiations .
Analyse Chemischer Reaktionen
Production Reactions of Mo-99
Mo-99 is synthesized through three principal methods, each involving distinct nuclear and chemical processes:
Nuclear Fission of Uranium-235
-
Reaction : 235U+n→99Mo+fission products+2−3n
Mo-99 is a fission product of neutron-irradiated uranium-235. This method dominates global production but relies on aging reactors and poses proliferation risks due to highly enriched uranium (HEU) usage . -
Specific Activity : High (carrier-free due to separation from uranium) .
Neutron Activation of Molybdenum-98
-
Reaction : 98Mo(n,γ)99Mo
Natural or enriched molybdenum targets are irradiated in reactors. While proliferation-resistant, this method yields lower specific activity due to isotopic dilution .
Accelerator-Driven Methods
-
Reaction : 100Mo(p,2n)99Tc→99Mo (indirect)
Proton bombardment of enriched Mo-100 produces Tc-99, which decays to Mo-99. This method avoids reactors but faces scalability challenges .
Table 1: Comparison of Mo-99 Production Methods
Decay Characteristics
Mo-99 decays via beta emission to Tc-99m, a metastable isomer:
99Moβ−99mTcγ99Tc
Chemical Behavior in Solution
Mo-99 exhibits versatile chemistry depending on its oxidation state (IV–VI) and environment:
Dissolution and Speciation
-
Alkaline Solutions : Forms molybdate ions (MoO42−) when dissolved in NaOH, enabling chromatographic separation .
-
Acidic Solutions : Generates polymeric oxyanions (e.g., Mo7O246−) at low pH, complicating purification .
Solvent Extraction
-
Di(2-ethylhexyl) Phosphoric Acid (D2EHPA) : Selectively extracts Mo-99 from uranium and fission products in HNO3, achieving >95% recovery .
-
Tributyl Phosphate (TBP) : Removes uranium via UO22+ complexation in nitric acid .
Table 2: Mo-99 Extraction Efficiency
Method | Recovery (%) | Key Contaminants Removed |
---|---|---|
D2EHPA + TBP | >95 | U, Sr, Ru, I |
Anion Exchange | 85–90 | Al, Fe, fission products |
Molten Salt | 70–80 | Fluoride salts, rare earths |
Behavior in Molten Salt Reactors (MSRs)
In MSRs, Mo-99 partitions into fluoride salts (e.g., FLiBe) as MoF62−. Key reactions include:
Challenges in Purification
Wissenschaftliche Forschungsanwendungen
Production of Molybdenum-99
Mo-99 is predominantly produced through the irradiation of uranium targets in nuclear reactors. The most common method involves using highly enriched uranium (HEU) or low-enriched uranium (LEU) targets. The fission of uranium generates Mo-99 along with other isotopes, which are then chemically separated for medical use. The half-life of Mo-99 is approximately 66 hours, allowing it to be transported to medical facilities where it decays into Tc-99m with a half-life of about 6 hours .
Table 1: Common Production Methods for Mo-99
Method | Description | Current Status |
---|---|---|
Nuclear Reactor Irradiation | Fission of uranium targets produces Mo-99. | Widely used |
Photo-fission | Utilizes high-intensity light beams on uranium to produce Mo-99. | Emerging technology |
Aqueous Homogeneous Reactor | A reactor technology using low-enriched uranium for Mo-99 production. | Investigational |
Clinical Applications
The primary application of Mo-99 is in the production of Tc-99m, which is used in over 30 million diagnostic procedures annually worldwide . The versatility and favorable properties of Tc-99m make it the most utilized radioisotope in nuclear medicine. Below are some key applications:
Diagnostic Imaging
Tc-99m is employed in various imaging techniques due to its short half-life and optimal photon energy (140 keV), which is ideal for detection by gamma cameras. Common imaging procedures include:
- Myocardial Perfusion Imaging: Assessing blood flow to the heart muscles.
- Bone Scans: Detecting skeletal metastases and other bone diseases.
- Renal Imaging: Evaluating kidney function and structure.
Cancer Detection and Management
Tc-99m plays a vital role in identifying cancerous tissues and monitoring treatment responses. Its ability to bind to specific biological molecules allows targeted imaging of tumors .
Research Applications
Mo-99 and its decay product Tc-99m are also used in various research settings, including studies on drug delivery systems, biological processes, and the development of new radiopharmaceuticals.
Case Study 1: Cardiac Stress Testing
In the United States, over 40,000 medical procedures involving Tc-99m are performed daily, with a significant portion dedicated to cardiac stress tests. These tests utilize Tc-99m to visualize blood flow in heart tissues, aiding in the diagnosis of coronary artery disease .
Case Study 2: Cancer Imaging
A notable application of Tc-99m is in bone scans for cancer patients. A study demonstrated that Tc-99m can effectively localize metastatic lesions, providing critical information for treatment planning and monitoring disease progression .
Challenges and Future Directions
Despite its widespread use, the production of Mo-99 faces several challenges:
- Supply Chain Vulnerabilities: The reliance on a limited number of reactors for Mo-99 production can lead to supply disruptions.
- Nuclear Proliferation Risks: The use of HEU poses significant proliferation concerns; thus, there is a push towards using LEU or alternative production methods.
- Emerging Technologies: Research into photo-fission and other innovative production methods aims to enhance the reliability and safety of Mo-99 supply chains .
Wirkmechanismus
The primary mechanism of action of Mo-99 is its decay to Tc-99m. Mo-99 decays by beta emission to produce Tc-99m, which then emits gamma radiation . This gamma radiation is detected by imaging devices to create detailed images of the body’s internal structures . The decay process involves the transformation of Mo-99 to Tc-99m, which is then eluted from a generator system using a saline solution .
Vergleich Mit ähnlichen Verbindungen
Key Properties of Mo-99
- Half-life : 66 hours (2.75 days) .
- Decay Pathway : β⁻ decay to Tc-99m (88.6% yield) .
- Production Methods : Primarily via neutron-induced fission of uranium-235 (U-235) in research reactors .
Supply Challenges
Mo-99 production faces global supply vulnerabilities due to reliance on aging reactors, geopolitical constraints, and radioactive decay during transport. For example, a 24-hour shipment delay reduces Mo-99 activity by 22% . Efforts to diversify production include transitioning from highly enriched uranium (HEU) to low-enriched uranium (LEU) targets to mitigate proliferation risks .
Comparison with Similar Compounds and Isotopes
Molybdenum-100 (Mo-100)
Mo-100 is a stable isotope of molybdenum used in cyclotron-based production of Tc-99m. Unlike reactor-produced Mo-99, Mo-100 undergoes neutron capture to form Mo-99, which then decays to Tc-99m.
Advantages of Mo-100 :
- Avoids uranium-based proliferation concerns.
- Enables decentralized production using medical cyclotrons .
- Companies like ASP Isotopes Inc. aim to commercialize Mo-100 enrichment by 2024 .
Limitations :
- Lower specific activity necessitates frequent target irradiation .
- High cost of enriched Mo-100 (~$1,000/gram) .
Technetium-99m (Tc-99m)
Tc-99m is the daughter product of Mo-99 and the workhorse of nuclear medicine. Its short half-life (6 hours) and ideal gamma emission make it unsuitable for direct production, necessitating Mo-99 generators .
Parameter | Mo-99 | Tc-99m |
---|---|---|
Half-life | 66 hours | 6 hours |
Decay Mode | β⁻ to Tc-99m | Isomeric transition (γ) |
Supply Chain Role | Parent isotope | Diagnostic agent |
Challenges :
- Tc-99m cannot be stockpiled due to its short half-life, requiring continuous Mo-99 production .
- Global Mo-99 shortages directly impact Tc-99m availability .
Co-Produced Isotopes: Iodine-131 (I-131) and Xenon-133 (Xe-133)
Mo-99 production via U-235 fission also yields medically useful isotopes like I-131 (thyroid therapy) and Xe-133 (lung imaging). However, these are often underutilized due to cost-effective alternatives .
Isotope | Half-life | Application | Production Yield |
---|---|---|---|
I-131 | 8 days | Thyroid cancer therapy | ~0.8% of fission yield |
Xe-133 | 5.2 days | Lung ventilation imaging | ~1.1% of fission yield |
Key Insight: While I-131 and Xe-133 are valuable, their recovery is often economically unviable compared to dedicated production routes .
Comparison of Production Methods
Reactor-Based Fission
Process : Irradiation of U-235 targets (HEU or LEU) in research reactors (e.g., ANSTO’s OPAL reactor) .
Challenges :
- HEU use raises proliferation concerns, driving shifts to LEU .
- Complex dissolution of targets generates long-lived waste .
Emerging Technologies
Q & A
Basic Research Questions
Q. What are the primary reactor-based methodologies for Mo-99 production, and how do they differ in target design and neutron flux utilization?
Mo-99 is traditionally produced via neutron irradiation of uranium-235 (U-235) or molybdenum targets in research reactors. Uranium-based fission (yielding ~6.1% Mo-99 per fission) requires dissolving irradiated U-235 targets, which generates high waste volumes . Molybdenum activation methods use natural or enriched Mo targets, reducing uranium dependency but producing lower specific activity Mo-99 . Target geometry optimization (e.g., adjusting dimensions to match neutron flux distribution) can enhance yield by up to 21.4% .
Q. What safety protocols are critical when integrating Mo-99 production into existing research reactors?
Safety analyses must account for neutron flux redistribution caused by Mo-99 production devices, which may alter core power profiles and impact fuel performance. For example, LEU (low-enriched uranium) fuel conversion studies require reassessing margins to safety, including thermal-hydraulic limits and shielding requirements . Contamination risks (e.g., Mo-99 "breakthrough" in Tc-99m generators) necessitate routine quality control via ICP spectrometry and adherence to USP XIX purity standards .
Advanced Research Questions
Q. How can accelerator-based Mo-99 production reconcile yield limitations with reduced radioactive waste?
Accelerators using photonuclear reactions (e.g., γ-induced fission of U-238) or proton bombardment of Mo-100 avoid uranium entirely, minimizing high-level waste. However, current yields are lower than reactor-based fission. Computational modeling of beamline configurations (e.g., high-power electron accelerators) and target irradiation homogeneity can optimize production rates . Recent experiments show that natural Mo activation in accelerators produces <10% of fission-derived Mo-99 per gram, necessitating advanced target enrichment strategies .
Q. What methodologies address contradictions in Mo-99 yield data between fission and activation production routes?
Discrepancies arise from differing specific activities: fission-derived Mo-99 has higher purity but requires complex separation, while activation methods yield lower-specific-activity Mo-99 with simpler processing. Researchers use isotopic dilution analysis (e.g., ICP spectrometry) to quantify stable Mo carriers in decayed samples, enabling accurate yield comparisons . Hybrid approaches, such as combining reactor irradiation with post-processing 3D-printed recycling systems, improve cost efficiency by reusing enriched Mo .
Q. How does target dissolution efficiency impact Mo-99 recovery and waste generation?
Traditional alkaline dissolution of U-235 targets achieves ~85–90% Mo-99 recovery but generates acidic waste containing fission products like I-131 and Sr-90. Advanced techniques, such as solvent extraction or column chromatography, improve recovery rates to >95% while reducing waste volume . Computational fluid dynamics (CFD) models are used to optimize dissolution parameters (e.g., temperature, agitation) for minimal uranium residue .
Q. Experimental Design & Data Analysis
Q. What experimental frameworks are used to validate Mo-99 production scalability in non-reactor systems?
Pilot-scale accelerator facilities employ iterative design-test cycles:
- Beamline diagnostics : Real-time monitoring of proton beam stability and target heating .
- Post-irradiation analysis : Gamma spectroscopy to quantify Mo-99/Tc-99m ratios and impurity profiles .
- Waste characterization : Neutron activation analysis (NAA) to classify radioactive byproducts .
Q. How can researchers mitigate biases in comparative studies of Mo-99 production methods?
- Controlled variables : Standardize irradiation time, neutron flux (n/cm²·s), and target composition.
- Blind testing : Use third-party labs for activity measurements to avoid instrumentation bias .
- Statistical tools : Apply ANOVA to compare yields across multiple production batches, accounting for decay corrections .
Q. Ethical & Methodological Considerations
Q. What ethical guidelines govern data sharing and reproducibility in Mo-99 research?
Researchers must:
- Disclose conflicts of interest (e.g., funding from isotope suppliers) .
- Archive raw data (e.g., irradiation logs, spectrometry results) for ≥5 years to enable independent verification .
- Avoid redundant publications by clearly differentiating incremental improvements (e.g., target design tweaks) from novel methodologies .
Q. Emerging Innovations
Q. Can additive manufacturing (3D printing) enhance Mo-99 recycling or target fabrication?
3D-printed components (e.g., high-purity Mo filters) enable efficient recovery of enriched Mo from spent targets, reducing reliance on costly (~$1,000/g) virgin material . Topology-optimized targets with lattice structures improve neutron capture efficiency by 15–20% in computational simulations .
Q. What role do molten salt reactors (MSRs) play in sustainable Mo-99 production?
MSRs offer continuous online processing of Mo-99 from liquid fuel, avoiding solid target dissolution. Preliminary models suggest MSRs could achieve 2–3× higher daily yields than conventional reactors, though challenges like corrosion-resistant materials remain unresolved .
Eigenschaften
CAS-Nummer |
14119-15-4 |
---|---|
Molekularformel |
Mo |
Molekulargewicht |
98.907707 g/mol |
IUPAC-Name |
molybdenum-99 |
InChI |
InChI=1S/Mo/i1+3 |
InChI-Schlüssel |
ZOKXTWBITQBERF-AKLPVKDBSA-N |
SMILES |
[Mo] |
Isomerische SMILES |
[99Mo] |
Kanonische SMILES |
[Mo] |
Synonyme |
99Mo radioisotope Mo-99 radioisotope Molybdenum-99 |
Herkunft des Produkts |
United States |
Haftungsausschluss und Informationen zu In-Vitro-Forschungsprodukten
Bitte beachten Sie, dass alle Artikel und Produktinformationen, die auf BenchChem präsentiert werden, ausschließlich zu Informationszwecken bestimmt sind. Die auf BenchChem zum Kauf angebotenen Produkte sind speziell für In-vitro-Studien konzipiert, die außerhalb lebender Organismen durchgeführt werden. In-vitro-Studien, abgeleitet von dem lateinischen Begriff "in Glas", beinhalten Experimente, die in kontrollierten Laborumgebungen unter Verwendung von Zellen oder Geweben durchgeführt werden. Es ist wichtig zu beachten, dass diese Produkte nicht als Arzneimittel oder Medikamente eingestuft sind und keine Zulassung der FDA für die Vorbeugung, Behandlung oder Heilung von medizinischen Zuständen, Beschwerden oder Krankheiten erhalten haben. Wir müssen betonen, dass jede Form der körperlichen Einführung dieser Produkte in Menschen oder Tiere gesetzlich strikt untersagt ist. Es ist unerlässlich, sich an diese Richtlinien zu halten, um die Einhaltung rechtlicher und ethischer Standards in Forschung und Experiment zu gewährleisten.